Synthesis, Properties of Biodegradable Poly(Butylene Succinate-Co-Butylene 2-Methylsuccinate) and Application for Sustainable Release
Total Page:16
File Type:pdf, Size:1020Kb
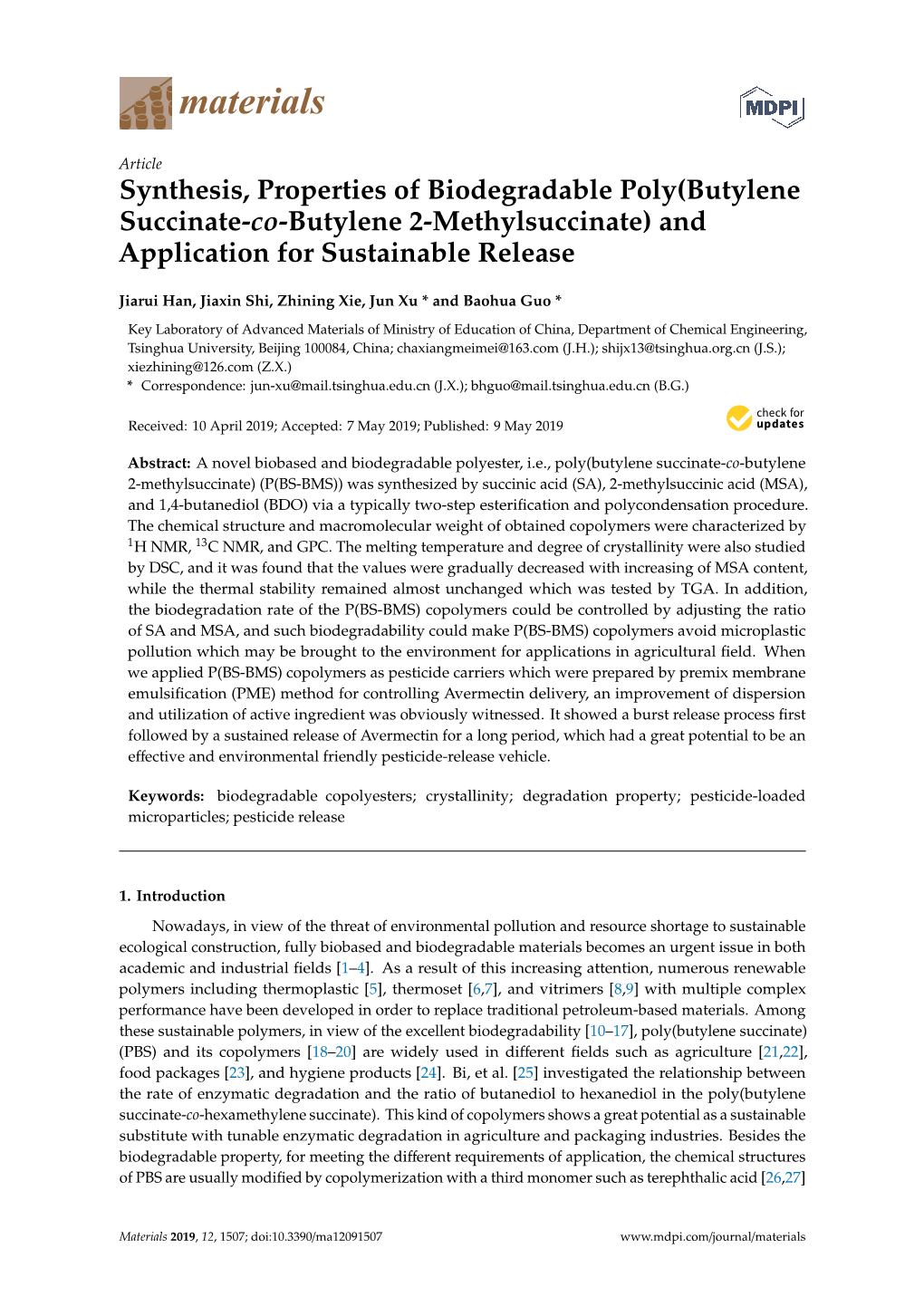
Load more
Recommended publications
-
Mitsubishi Chemical Sustainability Report 2018
Plant-Derived, Biodegradable Plastic BioPBS™ Relevant SDG SDG 12: Ensure sustainable consumption and production patterns Striving toward Sustainable Production We are now facing such global-scale risks as accelerating climate change, the depletion of natural resources, disparities in water resource distribution, expanding and graying populations, and food and agricultural issues. Given this critical situation, as a chemical company, we believe it is our mission to realize, through innovation, the efficient use of natural resources and energy, the utilization of renewable resources, and the reduction of environmental burden and to thereby enhance environmental and social sustainability. Initiatives to replace non-renewable petroleum with renewable biomass as the raw material for plastic produc- tion are helping to more efficiently use resources and greatly contribute to ensuring sustainable production, part of one of the SDGs. At the same time, making plastics biodegradable while retaining their useful proper- ties makes it easier for them to break down in the environment, helping to reduce environmental burden. With BioPBS™, a renewably sourced and biodegradable product, Mitsubishi Chemical (MCC) has developed a plastic that offers both of these unrelated attributes. Features of BioPBS™ Polybutylene succinate (PBS) is an aliphatic polyester resin made from succinic acid and 1,4-butanediol, two raw ingredients typically manufactured from petroleum. In contrast, BioPBS™ is made with succinic acid derived from plant materials, a renewable resource. Its excellent biodegradability at low temperatures—ulti- mately breaking down into water and CO2—sets it apart from other biodegradable plastics like polylactic acid (PLA) and polybutylene adipate terephthalate (PBAT). BioPBS™ also boasts such outstanding qualities as low-temperature heat sealability, compatibility with other materials, heat resistance and flexibility. -
Bio-Based and Biodegradable Plastics – Facts and Figures Focus on Food Packaging in the Netherlands
Bio-based and biodegradable plastics – Facts and Figures Focus on food packaging in the Netherlands Martien van den Oever, Karin Molenveld, Maarten van der Zee, Harriëtte Bos Rapport nr. 1722 Bio-based and biodegradable plastics - Facts and Figures Focus on food packaging in the Netherlands Martien van den Oever, Karin Molenveld, Maarten van der Zee, Harriëtte Bos Report 1722 Colophon Title Bio-based and biodegradable plastics - Facts and Figures Author(s) Martien van den Oever, Karin Molenveld, Maarten van der Zee, Harriëtte Bos Number Wageningen Food & Biobased Research number 1722 ISBN-number 978-94-6343-121-7 DOI http://dx.doi.org/10.18174/408350 Date of publication April 2017 Version Concept Confidentiality No/yes+date of expiration OPD code OPD code Approved by Christiaan Bolck Review Intern Name reviewer Christaan Bolck Sponsor RVO.nl + Dutch Ministry of Economic Affairs Client RVO.nl + Dutch Ministry of Economic Affairs Wageningen Food & Biobased Research P.O. Box 17 NL-6700 AA Wageningen Tel: +31 (0)317 480 084 E-mail: [email protected] Internet: www.wur.nl/foodandbiobased-research © Wageningen Food & Biobased Research, institute within the legal entity Stichting Wageningen Research All rights reserved. No part of this publication may be reproduced, stored in a retrieval system of any nature, or transmitted, in any form or by any means, electronic, mechanical, photocopying, recording or otherwise, without the prior permission of the publisher. The publisher does not accept any liability for inaccuracies in this report. 2 © Wageningen Food & Biobased Research, institute within the legal entity Stichting Wageningen Research Preface For over 25 years Wageningen Food & Biobased Research (WFBR) is involved in research and development of bio-based materials and products. -
Tacticity in Vinyl Polymers
TACTICITY IN VINYL POLYMERS Introduction Tacticity in polymers refers to a configurational order in molecular structures. Definition of polymer tacticity is properly given in a review article by Jenkins and co-workers (1), which reads The orderliness of the succession of configurational repeating units in the main chain of a regular macromolecule, a regular oligomer molecule, a regular block or a regular chain. Tacticity should not be confused with the conformational states of the poly- mer chains in space. The conformation refers to different arrangements of atoms and/or substituents of the polymer chain brought about by rotations about single bonds. Examples of different polymer conformations include the fully extended planar zig-zag, helical, folded chains, and random coils, etc. By contrast, the tac- tic configuration of the molecular chains refers to the organization of the atoms along the chain and configurational tactic isomerism involves the different struc- tural arrangements of the atoms and substituents in a polymer chain, which can be interconverted only by the breakage and reformation of primary chemi- cal bonds. There are three tactic forms in polymers: atactic, isotactic, and syn- diotactic. Isotactic and syndiotactic polymers are both stereoregular and thus are crystallizable. Atactic polymers, on the other hand, are usually completely amorphous, unless the side group is so small or highly polar as to permit crys- tallinity, e.g. poly(vinyl fluoride) (PVF) or polyacrylonitrile (PAN). Like some or- ganic compounds or naturally occurring polymers such as poly(L,D-lactic acid)s or poly(amino acid)s that differ in chirality, vinyl polymers differ in tacticity, which may be viewed as a pseudochirality form. -
Multi-Headed Chain Shuttling Agents and Their Use for the Preparation of Block Copolymers
(19) TZZ¥ ¥ T (11) EP 3 243 846 A2 (12) EUROPEAN PATENT APPLICATION (43) Date of publication: (51) Int Cl.: 15.11.2017 Bulletin 2017/46 C08F 4/659 (2006.01) C07F 3/02 (2006.01) C08F 10/00 (2006.01) C07F 3/06 (2006.01) (2006.01) (2006.01) (21) Application number: 17164138.4 C07F 5/02 C07F 5/06 (22) Date of filing: 20.07.2010 (84) Designated Contracting States: • Clark, Thomas AL AT BE BG CH CY CZ DE DK EE ES FI FR GB Midland, MI Michigan 48640 (US) GR HR HU IE IS IT LI LT LU LV MC MK MT NL NO • Frazier, Kevin PL PT RO SE SI SK SM TR Midland, MI Michigan 48642 (US) • Klamo, Sara (30) Priority: 29.07.2009 US 229610 P Midland, MI Michigan 48642 (US) • Timmers, Francis (62) Document number(s) of the earlier application(s) in Midland, MI Michigan 48642 (US) accordance with Art. 76 EPC: 10737196.5 / 2 459 598 (74) Representative: V.O. P.O. Box 87930 (71) Applicant: Dow Global Technologies Llc Carnegieplein 5 Midland, MI 48674 (US) 2508 DH Den Haag (NL) (72) Inventors: Remarks: • Arriola, Daniel This application was filed on 31-03-2017 as a Midland, MI Michigan 48642 (US) divisional application to the application mentioned under INID code 62. (54) MULTI-HEADED CHAIN SHUTTLING AGENTS AND THEIR USE FOR THE PREPARATION OF BLOCK COPOLYMERS (57) This disclosure relates to olefin polymerization shuttling agents (CSAs or MSAs) and for their use in pre- catalysts and compositions, their manufacture, and the paring blocky copolymers. -
A Glimpse of Biodegradable Polymers and Their Biomedical Applications
Spec. Matrices 2019; 7:1–19 Research Article Open Access Kazumasa Nomura* and Paul Terwilliger e-Polymers 2019; 19: 385–410 Self-dual Leonard pairs Review Article Open Access https://doi.org/10.1515/spma-2019-0001 Received May 8, 2018; accepted September 22, 2018 Tejas V. Shah and Dilip V. Vasava* Abstract: Let F denote a eld and let V denote a vector space over F with nite positive dimension. Consider A glimpse of biodegradablea pair A, A∗ of diagonalizable polymersF-linear maps and on V ,their each of which acts on an eigenbasis for the other one in an irreducible tridiagonal fashion. Such a pair is called a Leonard pair. We consider the self-dual case in which biomedical applicationsthere exists an automorphism of the endomorphism algebra of V that swaps A and A∗. Such an automorphism is unique, and called the duality A A∗. In the present paper we give a comprehensive description of this ↔ https://doi.org/10.1515/epoly-2019-0041 duality. In particular,NIPAM we - displayN-isopropylacrylamide an invertible F-linear map T on V such that the map X TXT− is the duality Received December 04, 2018; accepted March 29, 2019. → A A∗. We expressDEAMT -as N, a N-diethyl polynomial acrylamide in A and A∗. We describe how T acts on ags, decompositions, ↔ NVC - N-vinylcaprolactam Abstract: Over the past two decades, biodegradableand 24 bases for V. MVE - Methyl vinyl ether polymers (BPs) have been widely used in biomedical Keywords: LeonardPEO pair,- poly(ethylene tridiagonal matrix, oxide) self-dual applications such as drug carrier, gene delivery, tissue PPO - poly(propylene oxide) engineering, diagnosis, medical devices, and antibac- Classication: 17B37,15A21AAc - Acrylic acid terial/antifouling biomaterials. -
Analysis of Flammability and Smoke Emission of Rigid Polyurethane
J Therm Anal Calorim DOI 10.1007/s10973-017-6294-4 Analysis of flammability and smoke emission of rigid polyurethane foams modified with nanoparticles and halogen-free fire retardants 1 1 2 1 Kamila Salasinska • Monika Borucka • Milena Leszczyn´ska • Wojciech Zatorski • 1 1 2 Maciej Celin´ski • Agnieszka Gajek • Joanna Ryszkowska Received: 30 November 2016 / Accepted: 8 March 2017 Ó The Author(s) 2017. This article is an open access publication Abstract Using one-step method, rigid polyurethane Introduction foams were made, modified with developed fire retardant systems containing halogen-free flame retardants and Rigid polyurethane foams (RPUF) are used in many areas, nanofillers in the form of multi-walled carbon nanotubes or including construction industry as one of the best com- nanoscale titanium dioxide. The materials were subjected mercially available insulation materials. RPUF have very to a test using a cone calorimeter and smoke-generating good mechanical properties, resistance to aging and water chamber, and selected samples were further analyzed via and also atmospheric factors [1–4]. Unfortunately, rigid thermogravimetry and oxygen index. Moreover, the prod- polyurethane foams have also some disadvantages, among ucts of thermal degradation of selected samples were which special attention should be paid to flammability and identified using gas chromatography with mass spectrom- toxicity of the gas products emitted during thermal degra- eter. Conducted flammability tests confirmed the presence dation and combustion [5]. of a synergistic effect between the used nanofillers and Combustion of polymeric materials is an exothermic halogen-free flame retardants. It has been observed that the reaction of the catalytic oxidation of organic compounds carbonized layer, the formation of which favored the carried by energy supplied in the form of heat and forming presence of nanoadditives, inhibits the combustion process. -
Chain Structure Characterization
CHAIN STRUCTURE CHARACTERIZATION Gregory Beaucage and Amit S. Kulkarni Department of Chemical and Materials Engineering, University of Cincinnati, Cincinnati, Ohio 45221-0012 I. Introduction to Structure in Synthetic Macromolecules a) Dimensionality and Statistical Descriptions b) Chain Persistence and the Kuhn Unit c) Coil Structure and Chain Scaling Transitions d) Measures of Coil Size Rg and Rh II. Local Structure and its Ramifications a) Tacticity c) Branching d) Crystallization e) Hyperbranched Polymers III. Summary 1 I. Introduction to Structure in Synthetic Macromolecules a) Dimensionality and Statistical Descriptions Synthetic polymers display some physical characteristics that we can identify as native to this class of materials, particularly shear thinning rheology, rubber elasticity, and chain folded crystals. These properties are inherent to long-chain linear and weakly branched molecules and are not drastically different across a wide range of chemical make-ups. We can consider these features to define synthetic macromolecules as a distinct category of materials. The realization of this special category of materials necessitated the definition of a structural model broad enough to encompass nylon to polyethylene yet specific enough that detailed analytically available features could be used to define the major properties of interest, especially those native to this class of materials. This structural model for polymer chains is based on the random walk statistics observed by Robert Brown in studies of pollen grains and explained by Einstein in 1905. It is a trivial exercise to construct a random walk on a cubic lattice using a PC, Figure 1. From such a walk we can observe certain features of the general model for a polymer chain. -
Chem3020: Polymer Chemistry
CHEM3020: POLYMER CHEMISTRY Unit-5: Preparation, structure, properties and application of polymers Polyethylene, polystyrene and styrene copolymers Prof. Rafique Ul Islam Department of Chemistry, MGCU, Motihari CHEM3020: POLYMER CHEMISTRY UNIT-5 Polyethylene, polystyrene and styrene copolymers Polyethylene: The first commercial ethylene polymer was branched polyethylene commonly designated as Low density polyethylene. Polyethylene was first prepared using ethylene under 1400 atm pressure at 1700 C. Ethylene is made from the thermal and catalytic cracking of different hydrocarbons ranging from ethane obtained from natural gas to fuel oil. Presence of traces of oxygen initiate the polymerization process of ethylene readily. Rapid exothermic reaction can occur, and violent explosion have take place. Impurities present in the monomer, such as hydrogen and acetylene, may act as chain transfer agents and yield low molecular weight polyethylene. To obtained high molecular weight polymeric product, the imputiies must be carefully removed. Besides oxygen, peroxides, hydroperoxides and azo compounds can also be used as initiators for the polymerization process. Manufacturing: The manufacture of polyethylene follows addition polymerization kinetics involving catalysis of purified ethylene. Its melting point is 85–110oC. Under high pressure process, the density of polyethylene is 0.91-0.93 , whereas under low pressure the density is 0.96. CHEM3020: POLYMER CHEMISTRY UNIT-5 There are three ways by which polyethylene is manufactured – a. High Pressure Process : In this process, peroxide is used as a catalyst at 100-300oC and produces low density randomly oriented polymer of low melting point. The process is carried out at pressure of 1000–2500 atms. In this process Low Density Polyethylene (LDPE) is obtained. -
Performance Materials Solutions for the Polymer Industry Table of Contents Improving Well-Being by Offering the Best of Nature
PERFORMANCE MATERIALS SOLUTIONS FOR THE POLYMER INDUSTRY TABLE OF CONTENTS IMPROVING WELL-BEING BY OFFERING THE BEST OF NATURE Roquette is a global leader in plant-based ingredients, Roquette provides high performing, innovative and a pioneer of plant proteins and a leading provider of sustainable plant-based solutions for industrial pharmaceutical excipients. applications. In collaboration with its customers and partners, the group addresses current and future societal challenges by unlocking the potential of nature to offer the best ingredients for food, nutrition and health markets. These ingredients respond to unique and essential needs, enable healthier lifestyles and are critical components of life-saving medicines. Thanks to a constant drive for innovation and a long- term vision, the group is committed to improving the well-being of millions of people all over the world while taking care of resources and territories. Roquette currently operates in over 100 countries, has a turnover of around 3.7 billion euros and employs 8,670 people worldwide. Roquette is a monomer producer from 100% biomass feedstock to serve brand owners and consumers. ROQUETTE GROUP KEY FIGURES 100+countries served 5000+customers years85 of industrial 8670employees by ONE Global and operational Commercial Network excellence 45+nationalities industrial25 sites patents40 / year €3.7bn turnover 300+R&D workforce PRODUCTION EXPERTISE STARCH PRODUCTION SCHEME Native starch Drying STARCH = o po r f lyme ts glu ni Modification cose u Hydrolysis Enzymes Modified starch -
Tacticity Control of Polypropylene Using a C2-Symmetric Family of Catalysts
TACTICITY CONTROL OF POLYPROPYLENE USING A C2-SYMMETRIC FAMILY OF CATALYSTS A Thesis by NATHAN PRENTICE RIFE Submitted to the Office of Graduate Studies of Texas A&M University in partial fulfillment of the requirements for the degree of MASTER OF SCIENCE December 2007 Major Subject: Chemistry TACTICITY CONTROL OF POLYPROPYLENE USING A C2-SYMMETRIC FAMILY OF CATALYSTS A Thesis by NATHAN PRENTICE RIFE Submitted to the Office of Graduate Studies of Texas A&M University in partial fulfillment of the requirements for the degree of MASTER OF SCIENCE Approved by: Chair of Committee, Stephen A. Miller Committee Members, David E. Bergbreiter Jaime C. Grunlan Head of Department, David H. Russell December 2007 Major Subject: Chemistry iii ABSTRACT Tacticity Control of Polypropylene Using a C2-Symmetric Family of Catalysts. (December 2007) Nathan Prentice Rife, B.S., McMurry University Chair of Advisory Committee: Dr. Stephen A. Miller A family of C2-symmetric catalysts was designed and synthesized with the intent to polymerize propylene. The catalyst was designed to be C2-symmetric for the specific goal that the catalyst would have two identical sites for the propagation of the polymer and therefore eliminate some of the stereoerrors that occur in the propagation of the polymer chain. This catalyst would also operate under simple enantiomorphic site control and therefore the insertion of the monomer would be governed by the ligand surrounding the active site. The ligands were synthesized with increasing degrees of steric bulk with the intention to determine if a catalyst system could generate elastomeric polypropylene. Enantiomorphic site control polypropylene utilizes statistical methods to determine the Si and Re content of a given polymer chain as a function of the variable . -
Microplastic Extraction Protocols Can Impact the Polymer Structure
Pfohl et al. Microplastics and Nanoplastics (2021) 1:8 Microplastics and https://doi.org/10.1186/s43591-021-00009-9 Nanoplastics RESEARCH ARTICLE Open Access Microplastic extraction protocols can impact the polymer structure Patrizia Pfohl1, Christian Roth1, Lars Meyer1, Ute Heinemeyer1, Till Gruendling1, Christiane Lang1, Nikolaus Nestle1, Thilo Hofmann2, Wendel Wohlleben1 and Sarah Jessl1,3* Abstract Although microplastics are ubiquitous in today’s natural environments, our understanding of the materials, quantities, and particle sizes involved remains limited. The recovery of microplastics from different types of environmental matrices requires standardized matrix digestion protocols that allow inter-laboratory comparisons and that have no effect on the polymers themselves. A number of commonly used digestion methods rely on oxidation with concentrated hydrogen peroxide solutions to remove organic matter from the matrix. However, this can alter the nature of polymers through hydrolysis and often does not lead to a complete matrix removal. We have therefore investigated the use of two altered matrix digestion protocols, an acidic (Fenton) protocol and a new alkaline (Basic Piranha) protocol, focusing mainly on the effect on biodegradable polymers (polylactide, polybutylene adipate terephthalate, polybutylene succinate) and polymers with known degradation pathways via hydrolysis (thermoplastic polyurethanes, polyamide). Comparing the initial surface textures, chemical compositions, and particle size distributions with those obtained after -
Print This Article
PEER-REVIEWED ARTICLE bioresources.com Mimusops elengi Seed Shell Powder as a New Bio-Filler for Polypropylene-based Bio-Composites Mathialagan Muniyadi,a,* Tiffany Yit Siew Ng,a Yamuna Munusamy,a and Zhong Xian Ooi b Mimusops elengi seed shell powder (MESSP) was introduced as a new bio-filler in polypropylene (PP). The MESSP was characterized using a particle size analyzer, scanning electron microscopy (SEM), Fourier transform infrared spectroscopy, and a thermogravimetric analyzer. MESSP was successfully melt mixed with polypropylene to produce bio- composite at various MESSP loading. The processability and properties of the bio-composites were characterized by using processing torques, differential scanning calorimetry, tensile test, water absorption, and SEM. The processability of PP was not affected by the addition of MESSP, which was revealed from the minimum changes in the processing torques, melting temperature, crystallization temperature, and degree of crystallinity. The tensile strength and elastic modulus of the bio- composites were improved with an addition of MESSP of up to 10 wt.%. However, the elongation at break and resistance to water absorption decreased slightly with increased MESSP loading. Morphological observations revealed that the MESSP showed good dispersion and adhesion in the PP matrix of up to 5 wt.% MESSP. Above 5 wt.% MESSP, agglomerates formed, which influenced the physical- mechanical properties of the PP and MESSP bio-composites. Results indicated that PP/MESSP composites can be used to replace PP in