Morphologic Mapping of Lunar Impact Basins
Total Page:16
File Type:pdf, Size:1020Kb
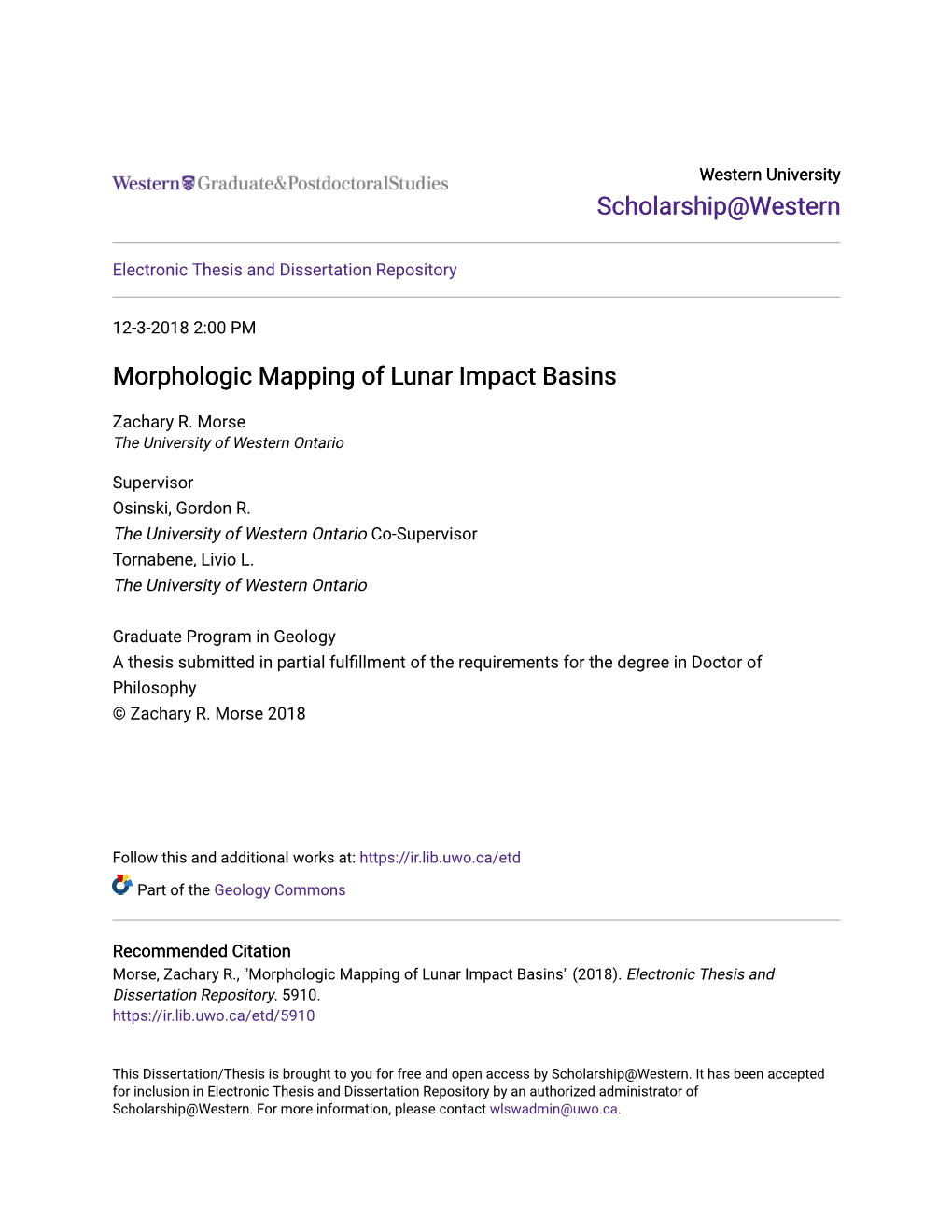
Load more
Recommended publications
-
Festskrift Til Harald Føsker Ellen Ekhaugen, Egil Larsen Og Fredrik Sjøli (Red.)
View metadata, citation and similar papers at core.ac.uk brought to you by CORE provided by NORA - Norwegian Open Research Archives Festskrift til Harald Føsker Ellen Ekhaugen, Egil Larsen og Fredrik Sjøli (red.) Kriminalomsorgens utdanningssenter, april 2014 1 Utgitt av Kriminalomsorgens utdanningssenter KRUS, 2014. Manusbearbeidelse og layout: Ottar Evensen og Maja Sørnes, KRUS Alle fotografier er gjengitt med artikkelforfatternes samtykke. ISBN trykket: 978-82-8257-033-6 ISBN pdf: 978-82-8257-034-3 Trykket hos 07-gruppen 2 Harald Føsker født 24. mai 1944 på Hamar Foto: Sturlason 3 BIDRAGSYTERE Balder, Jørgen – fhv uddannelseschef, KUC, Danmark Brucker, Hans-Jørgen – direktør, KRUS Five, Suzanne – seniorrådgiver, Kriminalomsorgsdirektoratet (KDI) Fridhov, Inger Marie – seniorrådgiver, Justisdepartementet Gislason, Gudmundur – fengselsdirektør/skoleleder, Island Hammerlin, Yngve – forsker, KRUS Hornslien, Anders - venn Johnsen, Berit – forskningsleder, KRUS Kornienko, Gennadij – generaldirektør, Den føderale russiske kriminalomsorgstjeneste Kristoffersen, Ragnar – forsker, KRUS Kvernvik Nilsen, Arne fengselsleder, Kriminalomsorgen Region Sør Larsen, Egil dekan, KRUS Lund-Isaksen, Erik – fhv. ekspedisjonssjef Nor, Susanne – HR direktør, Kriminalomsorgsdirektoratet (KDI) Orban, Franck – forsker, KRUS Porporino, Frank – Ph.D. Board Member ICPA Rentzmann, William – fhv. generaldirektør Kriminalforsorgen i Danmark Råen, Tore – friomsorgsleder i Nordtrøndelag Storberget, Knut – stortingsrepresentant og tidl. justisminister Vollan, Marianne -
SPHERE: the Exoplanet Imager for the Very Large Telescope J.-L
Astronomy & Astrophysics manuscript no. paper c ESO 2019 October 4, 2019 SPHERE: the exoplanet imager for the Very Large Telescope J.-L. Beuzit1; 2, A. Vigan2, D. Mouillet1, K. Dohlen2, R. Gratton3, A. Boccaletti4, J.-F. Sauvage2; 7, H. M. Schmid5, M. Langlois2; 8, C. Petit7, A. Baruffolo3, M. Feldt6, J. Milli13, Z. Wahhaj13, L. Abe11, U. Anselmi3, J. Antichi3, R. Barette2, J. Baudrand4, P. Baudoz4, A. Bazzon5, P. Bernardi4, P. Blanchard2, R. Brast12, P. Bruno18, T. Buey4, M. Carbillet11, M. Carle2, E. Cascone17, F. Chapron4, J. Charton1, G. Chauvin1; 23, R. Claudi3, A. Costille2, V. De Caprio17, J. de Boer9, A. Delboulbé1, S. Desidera3, C. Dominik15, M. Downing12, O. Dupuis4, C. Fabron2, D. Fantinel3, G. Farisato3, P. Feautrier1, E. Fedrigo12, T. Fusco7; 2, P. Gigan4, C. Ginski15; 9, J. Girard1; 14, E. Giro19, D. Gisler5, L. Gluck1, C. Gry2, T. Henning6, N. Hubin12, E. Hugot2, S. Incorvaia19, M. Jaquet2, M. Kasper12, E. Lagadec11, A.-M. Lagrange1, H. Le Coroller2, D. Le Mignant2, B. Le Ruyet4, G. Lessio3, J.-L. Lizon12, M. Llored2, L. Lundin12, F. Madec2, Y. Magnard1, M. Marteaud4, P. Martinez11, D. Maurel1, F. Ménard1, D. Mesa3, O. Möller-Nilsson6, T. Moulin1, C. Moutou2, A. Origné2, J. Parisot4, A. Pavlov6, D. Perret4, J. Pragt16, P. Puget1, P. Rabou1, J. Ramos6, J.-M. Reess4, F. Rigal16, S. Rochat1, R. Roelfsema16, G. Rousset4, A. Roux1, M. Saisse2, B. Salasnich3, E. Santambrogio19, S. Scuderi18, D. Segransan10, A. Sevin4, R. Siebenmorgen12 C. Soenke12, E. Stadler1, M. Suarez12, D. Tiphène4, M. Turatto3, S. Udry10, F. Vakili11, L. B. F. M. Waters20; 15, L. -
Ices on Mercury: Chemistry of Volatiles in Permanently Cold Areas of Mercury’S North Polar Region
Icarus 281 (2017) 19–31 Contents lists available at ScienceDirect Icarus journal homepage: www.elsevier.com/locate/icarus Ices on Mercury: Chemistry of volatiles in permanently cold areas of Mercury’s north polar region ∗ M.L. Delitsky a, , D.A. Paige b, M.A. Siegler c, E.R. Harju b,f, D. Schriver b, R.E. Johnson d, P. Travnicek e a California Specialty Engineering, Pasadena, CA b Dept of Earth, Planetary and Space Sciences, University of California, Los Angeles, CA c Planetary Science Institute, Tucson, AZ d Dept of Engineering Physics, University of Virginia, Charlottesville, VA e Space Sciences Laboratory, University of California, Berkeley, CA f Pasadena City College, Pasadena, CA a r t i c l e i n f o a b s t r a c t Article history: Observations by the MESSENGER spacecraft during its flyby and orbital observations of Mercury in 2008– Received 3 January 2016 2015 indicated the presence of cold icy materials hiding in permanently-shadowed craters in Mercury’s Revised 29 July 2016 north polar region. These icy condensed volatiles are thought to be composed of water ice and frozen Accepted 2 August 2016 organics that can persist over long geologic timescales and evolve under the influence of the Mercury Available online 4 August 2016 space environment. Polar ices never see solar photons because at such high latitudes, sunlight cannot Keywords: reach over the crater rims. The craters maintain a permanently cold environment for the ices to persist. Mercury surface ices magnetospheres However, the magnetosphere will supply a beam of ions and electrons that can reach the frozen volatiles radiolysis and induce ice chemistry. -
Glossary Glossary
Glossary Glossary Albedo A measure of an object’s reflectivity. A pure white reflecting surface has an albedo of 1.0 (100%). A pitch-black, nonreflecting surface has an albedo of 0.0. The Moon is a fairly dark object with a combined albedo of 0.07 (reflecting 7% of the sunlight that falls upon it). The albedo range of the lunar maria is between 0.05 and 0.08. The brighter highlands have an albedo range from 0.09 to 0.15. Anorthosite Rocks rich in the mineral feldspar, making up much of the Moon’s bright highland regions. Aperture The diameter of a telescope’s objective lens or primary mirror. Apogee The point in the Moon’s orbit where it is furthest from the Earth. At apogee, the Moon can reach a maximum distance of 406,700 km from the Earth. Apollo The manned lunar program of the United States. Between July 1969 and December 1972, six Apollo missions landed on the Moon, allowing a total of 12 astronauts to explore its surface. Asteroid A minor planet. A large solid body of rock in orbit around the Sun. Banded crater A crater that displays dusky linear tracts on its inner walls and/or floor. 250 Basalt A dark, fine-grained volcanic rock, low in silicon, with a low viscosity. Basaltic material fills many of the Moon’s major basins, especially on the near side. Glossary Basin A very large circular impact structure (usually comprising multiple concentric rings) that usually displays some degree of flooding with lava. The largest and most conspicuous lava- flooded basins on the Moon are found on the near side, and most are filled to their outer edges with mare basalts. -
Chapter Two: the Astronomers and Extraterrestrials
Warning Concerning Copyright Restrictions The Copyright Law of the United States (Title 17, United States Code) governs the making of photocopies or other reproductions of copyrighted materials, Under certain conditions specified in the law, libraries and archives are authorized to furnish a photocopy or other reproduction, One of these specified conditions is that the photocopy or reproduction is not to be used for any purpose other than private study, scholarship, or research , If electronic transmission of reserve material is used for purposes in excess of what constitutes "fair use," that user may be liable for copyright infringement. • THE EXTRATERRESTRIAL LIFE DEBATE 1750-1900 The idea of a plurality of worlds from Kant to Lowell J MICHAEL]. CROWE University of Notre Dame TII~ right 0/ ,It, U,,;v"Jily 0/ Camb,idg4' to P'''''' a"d s,1I all MO""" of oooks WM grattlrd by H,rr,y Vlf(;ff I $J4. TM U,wNn;fyltas pritr"d and pu"fisllrd rOffti",.ously sincr J5U. Cambridge University Press Cambridge London New York New Rochelle Melbourne Sydney Published by the Press Syndicate of the University of Cambridge In lovi ng The Pirr Building, Trumpingron Srreer, Cambridge CB2. I RP Claire H 32. Easr 57th Streer, New York, NY 1002.2., U SA J 0 Sramford Road, Oakleigh, Melbourne 3166, Australia and Mi ha © Cambridge Univ ersiry Press 1986 firsr published 1986 Prinred in rh e Unired Srares of America Library of Congress Cataloging in Publication Data Crowe, Michael J. The exrrarerresrriallife debare '750-1900. Bibliography: p. Includes index. I. Pluraliry of worlds - Hisrory. -
A La Torre Aaker Aalbers Aaldert Aarmour Aaron
A LA TORRE ABDIE ABLEMAN ABRAMOWITCH AAKER ABE ABLES ABRAMOWITZ AALBERS ABEE ABLETSON ABRAMOWSKY AALDERT ABEEL ABLETT ABRAMS AARMOUR ABEELS ABLEY ABRAMSEN AARON ABEKE ABLI ABRAMSKI AARONS ABEKEN ABLITT ABRAMSON AARONSON ABEKING ABLOTT ABRAMZON AASEN ABEL ABNER ABRASHKIN ABAD ABELA ABNETT ABRELL ABADAM ABELE ABNEY ABREU ABADIE ABELER ABORDEAN ABREY ABALOS ABELES ABORDENE ABRIANI ABARCA ABELI ABOT ABRIL ABATE ABELIN ABOTS ABRLI ABB ABELL ABOTSON ABRUZZO ABBA ABELLA ABOTT ABSALOM ABBARCROMBIE ABELLE ABOTTS ABSALON ABBAS ABELLS ABOTTSON ABSHALON ABBAT ABELMAN ABRAHAM ABSHER ABBATE ABELS ABRAHAMER ABSHIRE ABBATIELLO ABELSON ABRAHAMI ABSOLEM ABBATT ABEMA ABRAHAMIAN ABSOLOM ABBAY ABEN ABRAHAMOF ABSOLON ABBAYE ABENDROTH ABRAHAMOFF ABSON ABBAYS ABER ABRAHAMOV ABSTON ABBDIE ABERCROMBIE ABRAHAMOVITZ ABT ABBE ABERCROMBY ABRAHAMOWICZ ABTS ABBEKE ABERCRUMBIE ABRAHAMS ABURN ABBEL ABERCRUMBY ABRAHAMS ABY ABBELD ABERCRUMMY ABRAHAMSEN ABYRCRUMBIE ABBELL ABERDEAN ABRAHAMSOHN ABYRCRUMBY ABBELLS ABERDEEN ABRAHAMSON AC ABBELS ABERDEIN ABRAHAMSSON ACASTER ABBEMA ABERDENE ABRAHAMY ACCA ABBEN ABERG ABRAHM ACCARDI ABBERCROMBIE ABERLE ABRAHMOV ACCARDO ABBERCROMMIE ABERLI ABRAHMOVICI ACE ABBERCRUMBIE ABERLIN ABRAHMS ACERO ABBERDENE ABERNATHY ABRAHMSON ACESTER ABBERDINE ABERNETHY ABRAM ACETO ABBERLEY ABERT ABRAMCHIK ACEVEDO ABBETT ABEYTA ABRAMCIK ACEVES ABBEY ABHERCROMBIE ABRAMI ACHARD ABBIE ABHIRCROMBIE ABRAMIN ACHENBACH ABBING ABIRCOMBIE ABRAMINO ACHENSON ABBIRCROMBIE ABIRCROMBIE ABRAMO ACHERSON ABBIRCROMBY ABIRCROMBY ABRAMOF ACHESON ABBIRCRUMMY ABIRCROMMBIE ABRAMOFF -
What Literature Knows: Forays Into Literary Knowledge Production
Contributions to English 2 Contributions to English and American Literary Studies 2 and American Literary Studies 2 Antje Kley / Kai Merten (eds.) Antje Kley / Kai Merten (eds.) Kai Merten (eds.) Merten Kai / What Literature Knows This volume sheds light on the nexus between knowledge and literature. Arranged What Literature Knows historically, contributions address both popular and canonical English and Antje Kley US-American writing from the early modern period to the present. They focus on how historically specific texts engage with epistemological questions in relation to Forays into Literary Knowledge Production material and social forms as well as representation. The authors discuss literature as a culturally embedded form of knowledge production in its own right, which deploys narrative and poetic means of exploration to establish an independent and sometimes dissident archive. The worlds that imaginary texts project are shown to open up alternative perspectives to be reckoned with in the academic articulation and public discussion of issues in economics and the sciences, identity formation and wellbeing, legal rationale and political decision-making. What Literature Knows The Editors Antje Kley is professor of American Literary Studies at FAU Erlangen-Nürnberg, Germany. Her research interests focus on aesthetic forms and cultural functions of narrative, both autobiographical and fictional, in changing media environments between the eighteenth century and the present. Kai Merten is professor of British Literature at the University of Erfurt, Germany. His research focuses on contemporary poetry in English, Romantic culture in Britain as well as on questions of mediality in British literature and Postcolonial Studies. He is also the founder of the Erfurt Network on New Materialism. -
Laser Filamentation Interaction with Materials for Spectroscopic Applications
University of Central Florida STARS Electronic Theses and Dissertations, 2004-2019 2012 Laser Filamentation Interaction With Materials For Spectroscopic Applications Matthew Weidman University of Central Florida Part of the Electromagnetics and Photonics Commons, and the Optics Commons Find similar works at: https://stars.library.ucf.edu/etd University of Central Florida Libraries http://library.ucf.edu This Doctoral Dissertation (Open Access) is brought to you for free and open access by STARS. It has been accepted for inclusion in Electronic Theses and Dissertations, 2004-2019 by an authorized administrator of STARS. For more information, please contact [email protected]. STARS Citation Weidman, Matthew, "Laser Filamentation Interaction With Materials For Spectroscopic Applications" (2012). Electronic Theses and Dissertations, 2004-2019. 2385. https://stars.library.ucf.edu/etd/2385 LASER FILAMENTATION INTERACTION WITH MATERIALS FOR SPECTROSCOPIC APPLICATIONS by MATTHEW R. WEIDMAN B.S. Oregon Institute of Technology, 2006 M.S. University of Central Florida, 2007 A dissertation submitted in partial fulfillment of the requirements for the degree of Doctor of Philosophy in the College of Optics and Photonics at the University of Central Florida Orlando, Florida Fall Term 2012 Major Professor: Martin Richardson ©2012 Matthew R. Weidman ii ABSTRACT Laser filamentation is a non-diffracting propagation regime consisting of an intense core that is surrounded by an energy reservoir. For laser ablation based spectroscopy techniques such as Laser Induced Breakdown Spectroscopy (LIBS), laser filamentation enables the remote delivery of high power density laser radiation at long distances. This work shows a quasi- constant filament-induced mass ablation along a 35 m propagation distance. -
A 1 Case-PR/ }*Rciofft.;Is Report
.A 1 case-PR/ }*rciofft.;is Report (a) This eruption site on Mauna Loa Volcano was the main source of the voluminous lavas that flowed two- thirds of the distance to the town of Hilo (20 km). In the interior of the lava fountains, the white-orange color indicates maximum temperatures of about 1120°C; deeper orange in both the fountains and flows reflects decreasing temperatures (<1100°C) at edges and the surface. (b) High winds swept the exposed ridges, and the filter cannister was changed in the shelter of a p^hoehoc (lava) ridge to protect the sample from gas contamination. (c) Because of the high temperatures and acid gases, special clothing and equipment was necessary to protect the eyes. nose, lungs, and skin. Safety features included military flight suits of nonflammable fabric, fuil-face respirators that are equipped with dual acidic gas filters (purple attachments), hard hats, heavy, thick-soled boots, and protective gloves. We used portable radios to keep in touch with the Hawaii Volcano Observatory, where the area's seismic activity was monitored continuously. (d) Spatter activity in the Pu'u O Vent during the January 1984 eruption of Kilauea Volcano. Magma visible in the circular conduit oscillated in a piston-like fashion; spatter was ejected to heights of 1 to 10 m. During this activity, we sampled gases continuously for 5 hours at the west edge. Cover photo: This aerial view of Kilauea Volcano was taken in April 1984 during overflights to collect gas samples from the plume. The bluish portion of the gas plume contained a far higher density of fine-grained scoria (ash). -
Sky and Telescope
SkyandTelescope.com The Lunar 100 By Charles A. Wood Just about every telescope user is familiar with French comet hunter Charles Messier's catalog of fuzzy objects. Messier's 18th-century listing of 109 galaxies, clusters, and nebulae contains some of the largest, brightest, and most visually interesting deep-sky treasures visible from the Northern Hemisphere. Little wonder that observing all the M objects is regarded as a virtual rite of passage for amateur astronomers. But the night sky offers an object that is larger, brighter, and more visually captivating than anything on Messier's list: the Moon. Yet many backyard astronomers never go beyond the astro-tourist stage to acquire the knowledge and understanding necessary to really appreciate what they're looking at, and how magnificent and amazing it truly is. Perhaps this is because after they identify a few of the Moon's most conspicuous features, many amateurs don't know where Many Lunar 100 selections are plainly visible in this image of the full Moon, while others require to look next. a more detailed view, different illumination, or favorable libration. North is up. S&T: Gary The Lunar 100 list is an attempt to provide Moon lovers with Seronik something akin to what deep-sky observers enjoy with the Messier catalog: a selection of telescopic sights to ignite interest and enhance understanding. Presented here is a selection of the Moon's 100 most interesting regions, craters, basins, mountains, rilles, and domes. I challenge observers to find and observe them all and, more important, to consider what each feature tells us about lunar and Earth history. -
11 Get Continuances
' 0# triton ti Vo'ume 12, Hum"" 6 University 01 (a'ilornia, San Diego FriJay, January 22, '''' Regent's Meeting UCNewspaper Guidelines Approved With Provisions Carl Neiburger UCSD Vice Chancellor of Student Affairs City Editor George Murphy agreed with Haskins in an The Regents' Committee on Educational interview with the TRITO TIMES . He said Policy approved guidelines for student that before the amendement it was presumed newspapers yesterday. The approval, however, that " the papers would be consistent with the included the understanding that a guidelines" which, he observed, they had helped representative delegated by the chancellor to write. He said that " the reversal (of this review each issue within 24 hours after publication for rule violations. The proposal will come before the full board today for final On Page 3 Interview with approval. WILSON RILES, Supt. of Public Regent John Canaday, who first brought the matter of student papers before the board , Instruction and New UC Regent. introduced the 24 hour provision as one of four provisions he desired to be amended to the guidelines submitted to the Regents. The other presumption ) is what I think is not really three provisions stated that " responsibility for necessary." However, he foresaw no change in the conduct of student publications is vested in administrative policy at UCSD, other than that UCSD Scientists mesmerize media representatives at conference on Tuesday. (story on page 2) the chancellor, that apparent violations of the someone will be required to read the TRfTON -
Astronomy and Astrophysics
THE DECADE OF DISCOVERY IN ASTRONOMY AND ASTROPHYSICS Astronomy and Astrophysics Survey Committee Board on Physics and Astronomy Commission on Physical Sciences, Mathematics, and Applications National Research Council NATIONAL ACADEMY PRESS Washington, D.C. 1991 NATIONAL ACADEMY PRESS • 2101 Constitution Avenue, NW • Washington, DC 20418 NOTICE: The project that is the subject of this report was approved by the Governing Board of the National Research Council, whose members are drawn from the councils of the National Academy of Sciences, the National Academy of Engineering, and the Institute of Medicine. The members of the committee responsible for the report were chosen for their special compe_nces and with regard for appropriate balance. This report has been reviewed by a group other than the authors according to procedures approved by a Report Review Committee consisting of members of the National Academy of Sciences, the National Academy of Engineering, and the Institute of Medicine. This project was supported by the Department of Energy under Grant No. DE-FGO5- 89ER40421, the National Aeronautics and Space Administration and the National Science Foundation under Grant No. AST-8901685, the Naval Research Laboratory under Contract No. N00173-90-M-9744, and the Smithsonian Institution under Purchase Order No. SF0022430000. Additional support was provided by the Maurice Ewing Earth and Planetary Sciences Fund of the National Academy of Sciences created through a gift from the Palisades Geophysical Institute, Inc., and an anonymous donor. Library of Congress Cataloging-in-Publication Data National Research Council (U.S.). Astronomy and Astrophysics Survey Committee. The decade of discovery in astronomy and astrophysics / Astronomy and Astrophysics Survey Committee, Board on Physics and Astronomy, Commission on Physical Sciences, Mathematics, and Applications, National Research Council.