Non-Baseload Operation in Nuclear Power Plants: Load Following and Frequency Control Modes of Flexible Operation No
Total Page:16
File Type:pdf, Size:1020Kb
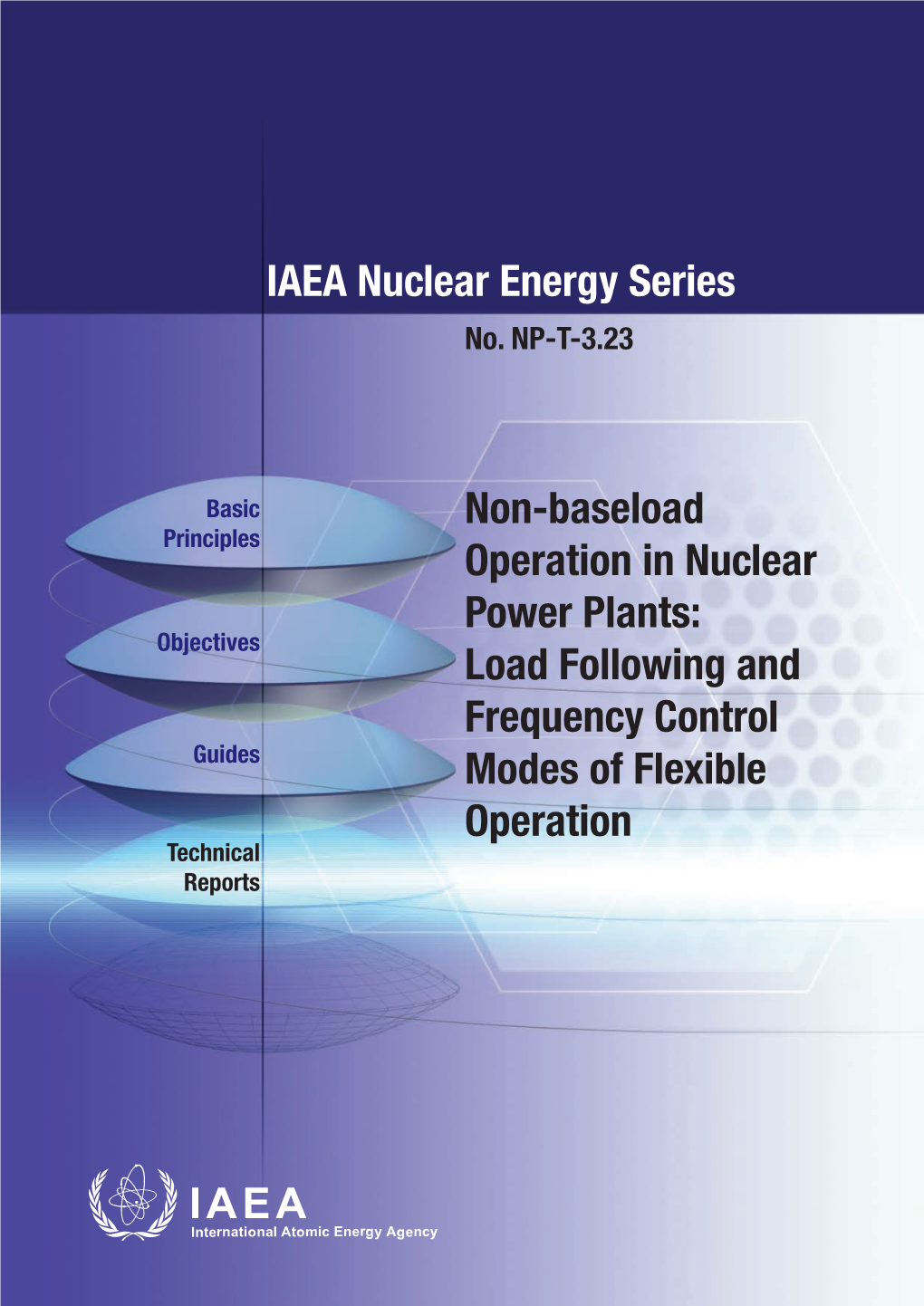
Load more
Recommended publications
-
Net Zero by 2050 a Roadmap for the Global Energy Sector Net Zero by 2050
Net Zero by 2050 A Roadmap for the Global Energy Sector Net Zero by 2050 A Roadmap for the Global Energy Sector Net Zero by 2050 Interactive iea.li/nzeroadmap Net Zero by 2050 Data iea.li/nzedata INTERNATIONAL ENERGY AGENCY The IEA examines the IEA member IEA association full spectrum countries: countries: of energy issues including oil, gas and Australia Brazil coal supply and Austria China demand, renewable Belgium India energy technologies, Canada Indonesia electricity markets, Czech Republic Morocco energy efficiency, Denmark Singapore access to energy, Estonia South Africa demand side Finland Thailand management and France much more. Through Germany its work, the IEA Greece advocates policies Hungary that will enhance the Ireland reliability, affordability Italy and sustainability of Japan energy in its Korea 30 member Luxembourg countries, Mexico 8 association Netherlands countries and New Zealand beyond. Norway Poland Portugal Slovak Republic Spain Sweden Please note that this publication is subject to Switzerland specific restrictions that limit Turkey its use and distribution. The United Kingdom terms and conditions are available online at United States www.iea.org/t&c/ This publication and any The European map included herein are without prejudice to the Commission also status of or sovereignty over participates in the any territory, to the work of the IEA delimitation of international frontiers and boundaries and to the name of any territory, city or area. Source: IEA. All rights reserved. International Energy Agency Website: www.iea.org Foreword We are approaching a decisive moment for international efforts to tackle the climate crisis – a great challenge of our times. -
Biodiversity Impacts Associated to Off- Shore Wind Power Projects
Bennun, L., van Bochove, J., Ng, C., Fletcher, C., Wilson, D., Phair, N., Carbone, G. (2021). Mitigating biodiversity impacts associated with solar and wind energy development. Guidelines for project developers. Gland, Switzerland: IUCN and Cambridge, UK: The Mitigating biodiversity impacts Biodiversity Consultancy. associated with solar and wind energy development Guidelines for project developers Biodiversity impacts associated to off- shore wind power IUCN GLOBAL BUSINESS AND BIODIVERSITY PROGRAMME projects The available scientific literature agrees on the key impacts of offshore wind: i) risk of collision mortality; ii) displacement due to disturbance (including noise impacts); iii) barrier effects (also including noise impacts); iv habitat loss; and v) indirect ecosystem-level effects. There is still much to understand on these five key impacts – but it is clear that that they must be considered carefully in all stages of offshore wind farm planning and development. The broad approach to undertaking an impact assessment for onshore wind energy is often equally relevant to offshore wind projects. There is also evidence that in some circumstances the wind farm area. Table 6-1 summarises the offshore wind farms can have positive biodiversity key biodiversity impacts of offshore wind farm impacts (case study 1), including introduction of new development, with selected references. For more habitat, artificial reef effects and a fishery ‘reserve detailed information, read the IUCN Mitigating effect’ where marine fauna tend to aggregate due biodiversity impacts associated with solar and to the exclusion of fishing (Section 7.2.1). However, wind energy development Guidelines for project it should be noted that this may in turn lead to developers. -
The Economic Potential of Three Nuclear-Renewable Hybrid Energy Systems Providing Thermal Energy to Industry
The Economic Potential of Three Nuclear-Renewable Hybrid Energy Systems Providing Thermal Energy to Industry Mark Ruth, Dylan Cutler, Francisco Flores-Espino, Greg Stark, and Thomas Jenkin National Renewable Energy Laboratory The Joint Institute for Strategic Energy Analysis is operated by the Alliance for Sustainable Energy, LLC, on behalf of the U.S. Department of Energy’s National Renewable Energy Laboratory, the University of Colorado-Boulder, the Colorado School of Mines, the Colorado State University, the Massachusetts Institute of Technology, and Stanford University. Technical Report NREL/TP-6A50-66745 December 2016 Contract No. DE-AC36-08GO28308 The Economic Potential of Three Nuclear-Renewable Hybrid Energy Systems Providing Thermal Energy to Industry Mark Ruth, Dylan Cutler, Francisco Flores-Espino, Greg Stark, and Thomas Jenkin National Renewable Energy Laboratory Prepared under Task No. SA15.1008 The Joint Institute for Strategic Energy Analysis is operated by the Alliance for Sustainable Energy, LLC, on behalf of the U.S. Department of Energy’s National Renewable Energy Laboratory, the University of Colorado-Boulder, the Colorado School of Mines, Colorado State University, the Massachusetts Institute of Technology, and Stanford University. JISEA® and all JISEA-based marks are trademarks or registered trademarks of the Alliance for Sustainable Energy, LLC. The Joint Institute for Technical Report Strategic Energy Analysis NREL/TP-6A50-66745 15013 Denver West Parkway December 2016 Golden, CO 80401 303-275-3000 • www.jisea.org Contract No. DE-AC36-08GO28308 NOTICE This report was prepared as an account of work sponsored by an agency of the United States government. Neither the United States government nor any agency thereof, nor any of their employees, makes any warranty, express or implied, or assumes any legal liability or responsibility for the accuracy, completeness, or usefulness of any information, apparatus, product, or process disclosed, or represents that its use would not infringe privately owned rights. -
Download Broschure
OUR CLIMATE PROCTECTION PROJECTS All commitments in Germany, Europe and all over the world. Content In the past years, the market for voluntary compensation for greenhouse gases has developed very dynamically. Above all, voluntary market (VER) Climate protection projects 4 11 good reasons for a voluntary 31 projects are being used to compensate CO2 emissions. compensation Energy efficiency Most of the certificates traded on the German market were generated by Climate protection projects 32 The Paradigm Project Healthy Cookstove renewable energy projects. In case of buyers, which are especially compa- 5 Forest protection / Afforestation and Water Treatment nies, they are compensated for their company footprints (CCF), product 33 Toyola Clean Cookstoves footprints (PCF) and travel. The quality of the projects is the most impor- 6 PROJECT TOGO 34 BioLite Homestove Project tant factor when buying a certificate. 8 Deutschland Plus Buyers see in a voluntary compensatory market an insufficient supply 35 AAC blocks manufacturing unit based on energy efficient technology of certificates from less developed countries, from Germany and from 10 Deutschland Plus Alpen Anaerobic digestion and heat generationat Sugar high-quality forest projects. 11 Deutschland Plus Schwarzwald 36 We as natureOffice too, see the greatest added value for climate and Corporation of Uganda 12 Deutschland Plus Rhön human beings in these projects and therefore focus our own projects on the themes: 13 Deutschland Plus Hunsrück Climate protection projects 37 14 Deutschland -
Case No COMP/M.2822- ENBW/ENI/GVS REGULATION (EEC)
EN Case No COMP/M.2822- ENBW/ENI/GVS Only the English text is available and authentic. REGULATION (EEC) No 4064/89 MERGER PROCEDURE Article 8 (2) Date: 17/12/2002 This text is made available for information purposes only and does not constitute an official publication. The official text of the decision will be published in the Official Journal of the European Communities. Commission Decision of 17/12/2002 declaring a concentration to be compatible with the common market and the EEA Agreement (Case No COMP/M.2822–EnBW / ENI / GVS) (Only the English text is authentic) (Text with EEA relevance) THE COMMISSION OF THE EUROPEAN COMMUNITIES, Having regard to the Treaty establishing the European Community, Having regard to the Agreement on the European Economic Area, and in particular Article 57(2)(a) thereof, Having regard to Council Regulation (EEC) No 4064/89 of 21 December 1989 on the control of concentrations between undertakings1, as last amended by Regulation (EC) No 1310/972, and in particular Article 8(2) and 22 (3) thereof, Having regard to the Commission's decision of 16 September 2002 to initiate proceedings in this case, Having regard to the opinion of the Advisory Committee on Concentrations3, Having regard to the final report of the hearing officer in this case4 1 OJ L 395, 30.12.1989, p. 1; corrected version in OJ L 257, 21.9.1990, p. 13. 2 OJ L 180, 9.7.1997, p. 1. 3 OJ C ...,...200. , p.... 4 … Commission européenne, B-1049 Bruxelles / Europese Commissie, B-1049 Brussel - Belgium. -
Nuclear Power Reactors in California
Nuclear Power Reactors in California As of mid-2012, California had one operating nuclear power plant, the Diablo Canyon Nuclear Power Plant near San Luis Obispo. Pacific Gas and Electric Company (PG&E) owns the Diablo Canyon Nuclear Power Plant, which consists of two units. Unit 1 is a 1,073 megawatt (MW) Pressurized Water Reactor (PWR) which began commercial operation in May 1985, while Unit 2 is a 1,087 MW PWR, which began commercial operation in March 1986. Diablo Canyon's operation license expires in 2024 and 2025 respectively. California currently hosts three commercial nuclear power facilities in various stages of decommissioning.1 Under all NRC operating licenses, once a nuclear plant ceases reactor operations, it must be decommissioned. Decommissioning is defined by federal regulation (10 CFR 50.2) as the safe removal of a facility from service along with the reduction of residual radioactivity to a level that permits termination of the NRC operating license. In preparation for a plant’s eventual decommissioning, all nuclear plant owners must maintain trust funds while the plants are in operation to ensure sufficient amounts will be available to decommission their facilities and manage the spent nuclear fuel.2 Spent fuel can either be reprocessed to recover usable uranium and plutonium, or it can be managed as a waste for long-term ultimate disposal. Since fuel re-processing is not commercially available in the United States, spent fuel is typically being held in temporary storage at reactor sites until a permanent long-term waste disposal option becomes available.3 In 1976, the state of California placed a moratorium on the construction and licensing of new nuclear fission reactors until the federal government implements a solution to radioactive waste disposal. -
Advantages of Applying Large-Scale Energy Storage for Load-Generation Balancing
energies Article Advantages of Applying Large-Scale Energy Storage for Load-Generation Balancing Dawid Chudy * and Adam Le´sniak Institute of Electrical Power Engineering, Lodz University of Technology, Stefanowskiego Str. 18/22, PL 90-924 Lodz, Poland; [email protected] * Correspondence: [email protected] Abstract: The continuous development of energy storage (ES) technologies and their wider utiliza- tion in modern power systems are becoming more and more visible. ES is used for a variety of applications ranging from price arbitrage, voltage and frequency regulation, reserves provision, black-starting and renewable energy sources (RESs), supporting load-generation balancing. The cost of ES technologies remains high; nevertheless, future decreases are expected. As the most profitable and technically effective solutions are continuously sought, this article presents the results of the analyses which through the created unit commitment and dispatch optimization model examines the use of ES as support for load-generation balancing. The performed simulations based on various scenarios show a possibility to reduce the number of starting-up centrally dispatched generating units (CDGUs) required to satisfy the electricity demand, which results in the facilitation of load-generation balancing for transmission system operators (TSOs). The barriers that should be encountered to improving the proposed use of ES were also identified. The presented solution may be suitable for further development of renewables and, in light of strict climate and energy policies, may lead to lower utilization of large-scale power generating units required to maintain proper operation of power systems. Citation: Chudy, D.; Le´sniak,A. Keywords: load-generation balancing; large-scale energy storage; power system services modeling; Advantages of Applying Large-Scale power system operation; power system optimization Energy Storage for Load-Generation Balancing. -
Monitoring Report 2019
Report Monitoring report 2019 BUNDESNETZAGENTUR | BUNDESKARTELLAMT | 1 Monitoring report 2019 in accordance with section 63(3) in conjunction with section 35 of the Energy Industry Act (EnWG) and section 48(3) in conjunction with section 53(3) of the Competition Act (GWB) Editorial deadline: 27 November 2019 2 | BUNDESNETZAGENTUR | BUNDESKARTELLAMT Bundesnetzagentur für Elektrizität, Gas, Bundeskartellamt Telekommunikation, Post und Eisenbahnen Referat 603 Arbeitsgruppe Energie-Monitoring Tulpenfeld 4 Kaiser-Friedrich-Straße 16 53113 Bonn 53113 Bonn [email protected] [email protected] BUNDESNETZAGENTUR | BUNDESKARTELLAMT | 3 German Energy Industry Act section 63(3) Reporting (3) Once a year, the Bundesnetzagentur shall publish a report on its activities and in agreement with the Bundeskartellamt, to the extent that aspects of competition are concerned, on the results of its monitoring activities, and shall submit the report to the European Commission and the Agency for the Cooperation of Energy Regulators (ACER). The report shall include the report by the Bundeskartellamt on the results of its monitoring activities under section 48(3) in conjunction with section 53(3) of the Competition Act as prepared in agreement with the Bundesnetzagentur to the extent that aspects of regulation of the distribution networks are concerned. The report shall include general instructions issued by the Federal Ministry of Economic Affairs and Energy in accordance with section 61. German Competition Act section 53(3) Activity report and monitoring reports (3) At least every two years, as part of its monitoring activities pursuant to section 48(3) sentence 1, the Bundeskartellamt shall prepare a report on the competitive conditions in the electricity generation market. -
Nuclear Power Plant Organization and Staffing for Lessons Learned
IAEA-TECDOC-1052 minium XA9848504 Nuclear power plant organization staffingand for improved performance: lessons learned INTERNATIONAL ATOMIC ENERGY AGENCY fl/U Lr\^ The originating Section of this publication in the IAEA was: Nuclear Power Engineering Section International Atomic Energy Agency Wagramer Strasse5 P.O. Box 100 A-1400 Vienna, Austria NUCLEAR POWER PLANT ORGANIZATION AND STAFFING FOR IMPROVED PERFORMANCE: LESSONS LEARNED IAEA, VIENNA, 1998 IAEA-TECDOC-1052 ISSN 1011-4289 ©IAEA, 1998 Printe IAEe th AustriAn y i d b a November 1998 The IAEA does not normally maintain stocks of reports in this series. However, microfiche copies of these reports can be obtained from INIS Clearinghouse International Atomic Energy Agency Wagramerstrasse5 0 10 P.Ox Bo . A-1400 Vienna, Austria Orders shoul accompaniee db prepaymeny db f Austriao t n Schillings 100, fore for e chequa th f mth IAEmf o n i n o i r eAo microfiche service coupons which may be ordered separately from the INIS Clearinghouse. FOREWORD Experience from well operated nuclear power plants (NPPs) aroun worle dth d indicates that an organizational structure that effectively supports plant operation s i essentias n i l economically achieving high level f safet so operationa d yan l performance same th t e A .time , in many Member States, energy market e beinar s g opene o competitiont d n i s i t I . consideration of this new competitive energy market that this publication focuses on organization and staffing of NPPs to improve efficiency and effectiveness. This publication is primarily intended for senior NPP and utility managers. -
038 12 Conduct of Operations
TMI-1 UFSAR CHAPTER 12 – CONDUCT OF OPERATIONS TABLE OF CONTENTS SECTION TITLE 12.0 CONDUCT OF OPERATIONS 12.1 ORGANIZATIONAL STRUCTURE 12.1.1 OFFSITE ORGANIZATION 12.1.2 OPERATING ORGANIZATION 12.1.2.1 PLANT DIVISION 12.1.2.1.1 OPERATIONS DEPARTMENT 12.1.2.1.2 MAINTENANCE DEPARTMENT 12.1.2.1.3 WORK MANAGEMENT DEPARTMENT 12.1.2.1.4 RADIOLOGICAL HEALTH AND SAFETY DEPARTMENT 12.1.2.1.5 CHEMISTRY DEPARTMENT 12.1.2.2 SECURITY DEPARTMENT 12.1.2.3 SITE ENGINEERING DEPARTMENT 12.1.2.3.1 PLANT ENGINEERING SECTION 12.1.2.3.2 DESIGN ENGINEERING SECTION 12.1.2.3.3 PROGRAMS SECTION 12.1.2.4 TRAINING DEPARTMENT 12.1.2.5 BUSINESS OPERATIONS DEPARTMENT 12.1.2.6 REGULATORY ASSURANCE DEPARTMENT 12.1.2.7 MANAGER – HUMAN RESOURCES 12.1.2.8 PROJECT MANAGEMENT DEPARTMENT 12.1.3 QUALIFICATION OF NUCLEAR PLANT PERSONNEL 12.1.4 SAFETY REVIEWS 12.2 TRAINING 12.2.1 TRAINING PROGRAMS 12.2.2 TECHNICAL TRAINING 12.2.2.1 MAINTENANCE TRAINING 12.2.2.2 RADIOLOGICAL CONTROLS/CHEMISTRY TRAINING PROGRAMS 12.2.3 OPERATIONS TRAINING 12.2.3.1 REPLACEMENT OPERATOR TRAINING PROGRAM (AO/CRO/SRO) 12.2.3.2 LICENSED OPERATOR REQUALIFICATION TRAINING PROGRAM (CRO/SRO) 12.2.3.3 SHIFT TECHNICAL ADVISOR (STA) TRAINING PROGRAM 12.2.3.4 AUXILIARY OPERATOR (AO) REQUALIFICATION TRAINING PROGRAM 12.2.4 TRAINING SUPPORT 12.2.4.1 GENERAL EMPLOYEE TRAINING PROGRAMS 12.2.4.2 FIRE PROTECTION TRAINING PROGRAM 12.2.4.3 EMERGENCY PREPAREDNESS TRAINING PROGRAMS 12.2.4.4 NOT USED 12.2.5 ENGINEERING SUPPORT PERSONNEL (ESP)TRAINING PROGRAM 12.2.6 TRAINING RECORDS CHAPTER 12 12-i REV. -
Policy Brief Organisation for Economic Co-Operation and Development
OCTOBER 2008Policy Brief ORGANISATION FOR ECONOMIC CO-OPERATION AND DEVELOPMENT Nuclear Energy Today Can nuclear Introduction energy help make Nuclear energy has been used to produce electricity for more than half a development century. It currently provides about 15% of the world’s supply and 22% in OECD sustainable? countries. The oil crisis of the early 1970s provoked a surge in nuclear power plant orders How safe is and construction, but as oil prices stabilised and even dropped, and enough nuclear energy? electricity generating plants came into service to meet demand, orders tailed off. Accidents at Three Mile Island in the United States (1979) and at Chernobyl How best to deal in Ukraine (1986) also raised serious questions in the public mind about nuclear with radioactive safety. waste? Now nuclear energy is back in the spotlight as many countries reassess their energy policies in the light of concerns about future reliance on fossil fuels What is the future and ageing energy generation facilities. Oil, coal and gas currently provide of nuclear energy? around two-thirds of the world’s energy and electricity, but also produce the greenhouse gases largely responsible for global warming. At the same For further time, world energy demand is expected to rise sharply in the next 50 years, information presenting all societies worldwide with a real challenge: how to provide the energy needed to fuel economic growth and improve social development while For further reading simultaneously addressing environmental protection issues. Recent oil price hikes, blackouts in North America and Europe and severe weather events have Where to contact us? also focused attention on issues such as long-term price stability, the security of energy supply and sustainable development. -
Incorporating Renewables Into the Electric Grid: Expanding Opportunities for Smart Markets and Energy Storage
INCORPORATING RENEWABLES INTO THE ELECTRIC GRID: EXPANDING OPPORTUNITIES FOR SMART MARKETS AND ENERGY STORAGE June 2016 Contents Executive Summary ....................................................................................................................................... 2 Introduction .................................................................................................................................................. 5 I. Technical and Economic Considerations in Renewable Integration .......................................................... 7 Characteristics of a Grid with High Levels of Variable Energy Resources ................................................. 7 Technical Feasibility and Cost of Integration .......................................................................................... 12 II. Evidence on the Cost of Integrating Variable Renewable Generation ................................................... 15 Current and Historical Ancillary Service Costs ........................................................................................ 15 Model Estimates of the Cost of Renewable Integration ......................................................................... 17 Evidence from Ancillary Service Markets................................................................................................ 18 Effect of variable generation on expected day-ahead regulation mileage......................................... 19 Effect of variable generation on actual regulation mileage ..............................................................