Impact of Peroxisome Proliferator-Activated Receptor-Α On
Total Page:16
File Type:pdf, Size:1020Kb
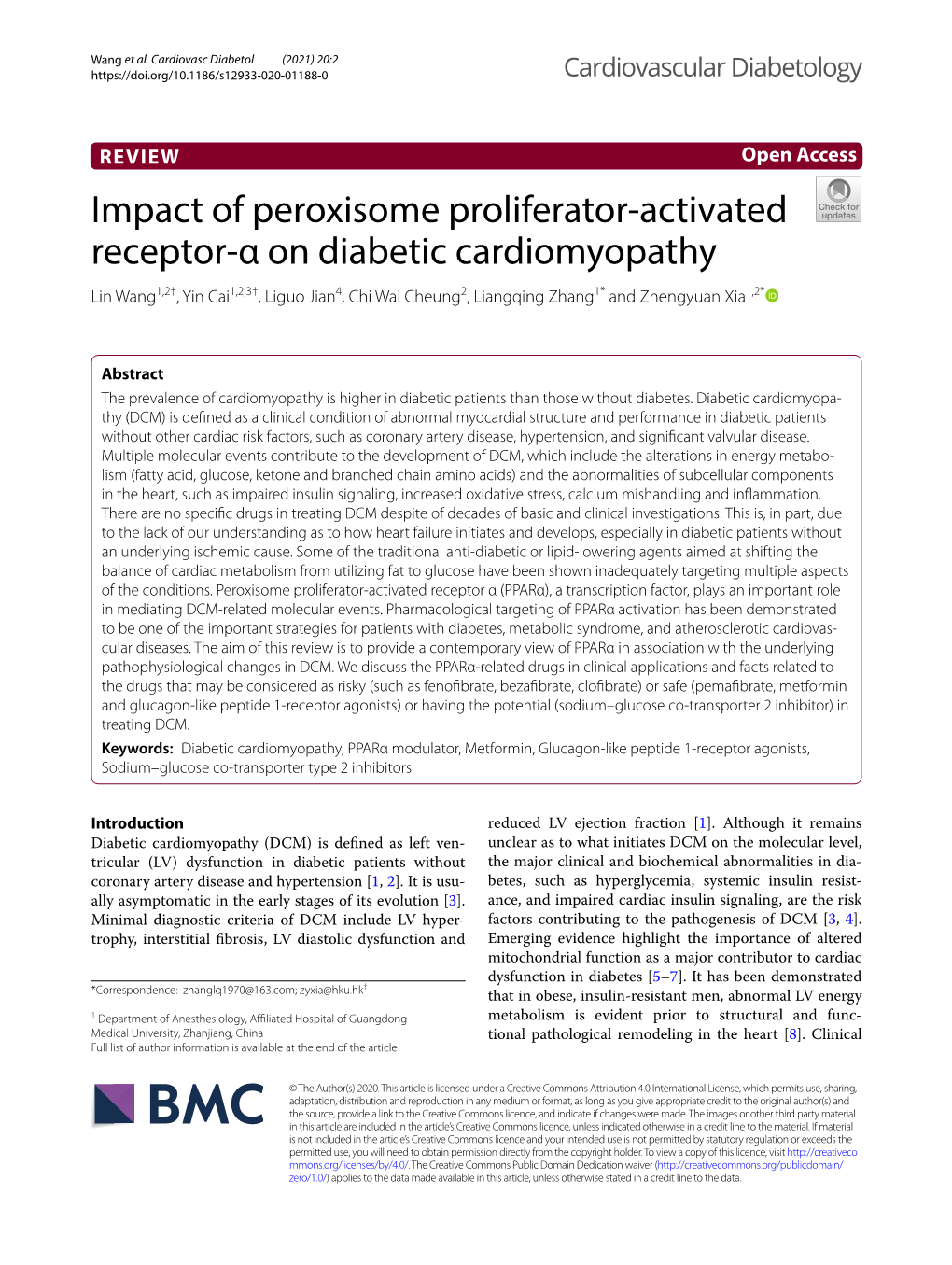
Load more
Recommended publications
-
Classification Decisions Taken by the Harmonized System Committee from the 47Th to 60Th Sessions (2011
CLASSIFICATION DECISIONS TAKEN BY THE HARMONIZED SYSTEM COMMITTEE FROM THE 47TH TO 60TH SESSIONS (2011 - 2018) WORLD CUSTOMS ORGANIZATION Rue du Marché 30 B-1210 Brussels Belgium November 2011 Copyright © 2011 World Customs Organization. All rights reserved. Requests and inquiries concerning translation, reproduction and adaptation rights should be addressed to [email protected]. D/2011/0448/25 The following list contains the classification decisions (other than those subject to a reservation) taken by the Harmonized System Committee ( 47th Session – March 2011) on specific products, together with their related Harmonized System code numbers and, in certain cases, the classification rationale. Advice Parties seeking to import or export merchandise covered by a decision are advised to verify the implementation of the decision by the importing or exporting country, as the case may be. HS codes Classification No Product description Classification considered rationale 1. Preparation, in the form of a powder, consisting of 92 % sugar, 6 % 2106.90 GRIs 1 and 6 black currant powder, anticaking agent, citric acid and black currant flavouring, put up for retail sale in 32-gram sachets, intended to be consumed as a beverage after mixing with hot water. 2. Vanutide cridificar (INN List 100). 3002.20 3. Certain INN products. Chapters 28, 29 (See “INN List 101” at the end of this publication.) and 30 4. Certain INN products. Chapters 13, 29 (See “INN List 102” at the end of this publication.) and 30 5. Certain INN products. Chapters 28, 29, (See “INN List 103” at the end of this publication.) 30, 35 and 39 6. Re-classification of INN products. -
Pemafibrate, a Novel Selective Peroxisome Proliferator-Activated
www.nature.com/scientificreports OPEN Pemafibrate, a novel selective peroxisome proliferator-activated receptor alpha modulator, Received: 25 July 2016 Accepted: 11 January 2017 improves the pathogenesis in Published: 14 February 2017 a rodent model of nonalcoholic steatohepatitis Yasushi Honda1, Takaomi Kessoku1, Yuji Ogawa1, Wataru Tomeno1, Kento Imajo1, Koji Fujita1, Masato Yoneda1, Toshiaki Takizawa2, Satoru Saito1, Yoji Nagashima3 & Atsushi Nakajima1 The efficacy of peroxisome proliferator-activated receptorα -agonists (e.g., fibrates) against nonalcoholic fatty liver disease (NAFLD)/nonalcoholic steatohepatitis (NASH) in humans is not known. Pemafibrate is a novel selective peroxisome proliferator-activated receptorα modulator that can maximize the beneficial effects and minimize the adverse effects of fibrates used currently. In a phase-2 study, pemafibrate was shown to improve liver dysfunction in patients with dyslipidaemia. In the present study, we first investigated the effect of pemafibrate on rodent models of NASH. Pemafibrate efficacy was assessed in a diet-induced rodent model of NASH compared with fenofibrate. Pemafibrate and fenofibrate improved obesity, dyslipidaemia, liver dysfunction, and the pathological condition of NASH. Pemafibrate improved insulin resistance and increased energy expenditure significantly. To investigate the effects of pemafibrate, we analysed the gene expressions and protein levels involved in lipid metabolism. We also analysed uncoupling protein 3 (UCP3) expression. Pemafibrate stimulated lipid turnover and upregulated UCP3 expression in the liver. Levels of acyl-CoA oxidase 1 and UCP3 protein were increased by pemafibrate significantly. Pemafibrate can improve the pathogenesis of NASH by modulation of lipid turnover and energy metabolism in the liver. Pemafibrate is a promising therapeutic agent for NAFLD/NASH. The incidence of nonalcoholic fatty liver disease (NAFLD) is increasing worldwide. -
A61p1/16 (2006.01) A61p3/00 (2006.01) Km, Ml, Mr, Ne, Sn, Td, Tg)
( (51) International Patent Classification: TR), OAPI (BF, BJ, CF, CG, Cl, CM, GA, GN, GQ, GW, A61P1/16 (2006.01) A61P3/00 (2006.01) KM, ML, MR, NE, SN, TD, TG). A61K 31/192 (2006.01) C07C 321/28 (2006.01) Declarations under Rule 4.17: (21) International Application Number: — as to the applicant's entitlement to claim the priority of the PCT/IB2020/000808 earlier application (Rule 4.17(iii)) (22) International Filing Date: Published: 25 September 2020 (25.09.2020) — with international search report (Art. 21(3)) (25) Filing Language: English — before the expiration of the time limit for amending the claims and to be republished in the event of receipt of (26) Publication Language: English amendments (Rule 48.2(h)) (30) Priority Data: 62/906,288 26 September 2019 (26.09.2019) US (71) Applicant: ABIONYX PHARMA SA [FR/FR] ; 33-43 Av¬ enue Georges Pompidou, Batiment D, 31130 Bahna (FR). (72) Inventor: DASSEUX, Jean-Louis, Henri; 7 Allees Charles Malpel, Bat. B, 31300 Toulouse (FR). (74) Agent: HOFFMANN EITLE PATENT- UND RECHTSANWALTE PARTMBB, ASSOCIATION NO. 151; Arabellastrasse 30, 81925 Munich (DE). (81) Designated States (unless otherwise indicated, for every kind of national protection available) : AE, AG, AL, AM, AO, AT, AU, AZ, BA, BB, BG, BH, BN, BR, BW, BY, BZ, CA, CH, CL, CN, CO, CR, CU, CZ, DE, DJ, DK, DM, DO, DZ, EC, EE, EG, ES, FI, GB, GD, GE, GH, GM, GT, HN, HR, HU, ID, IL, IN, IR, IS, IT, JO, JP, KE, KG, KH, KN, KP, KR, KW, KZ, LA, LC, LK, LR, LS, LU, LY, MA, MD, ME, MG, MK, MN, MW, MX, MY, MZ, NA, NG, NI, NO, NZ, OM, PA, PE, PG, PH, PL, PT, QA, RO, RS, RU, RW, SA, SC, SD, SE, SG, SK, SL, ST, SV, SY, TH, TJ, TM, TN, TR, TT, TZ, UA, UG, US, UZ, VC, VN, WS, ZA, ZM, ZW. -
Effects of Pemafibrate on Glucose Metabolism Markers and Liver
Yokote et al. Cardiovasc Diabetol (2021) 20:96 https://doi.org/10.1186/s12933-021-01291-w Cardiovascular Diabetology ORIGINAL INVESTIGATION Open Access Efects of pemafbrate on glucose metabolism markers and liver function tests in patients with hypertriglyceridemia: a pooled analysis of six phase 2 and phase 3 randomized double‐blind placebo‐controlled clinical trials Koutaro Yokote1,2*, Shizuya Yamashita3, Hidenori Arai4, Eiichi Araki5, Mitsunori Matsushita6, Toshiaki Nojima7, Hideki Suganami7 and Shun Ishibashi8 Abstract Background: Increased risk of cardiovascular events is associated not only with dyslipidemias, but also with abnor- malities in glucose metabolism and liver function. This study uses pooled analysis to explore the in-depth efects of pemafbrate, a selective peroxisome proliferator-activated receptor α modulator (SPPARMα) already known to decrease elevated triglycerides, on glucose metabolism and liver function in patients with hypertriglyceridemia. Methods: We performed a post-hoc analysis of six phase 2 and phase 3 Japanese randomized double-blind placebo- controlled trials that examined the efects of daily pemafbrate 0.1 mg, 0.2 mg, and 0.4 mg on glucose metabolism markers and liver function tests (LFTs). Primary endpoints were changes in glucose metabolism markers and LFTs from baseline after 12 weeks of pemafbrate treatment. All adverse events and adverse drug reactions were recorded as safety endpoints. Results: The study population was 1253 patients randomized to placebo (n 298) or pemafbrate 0.1 mg/day (n 127), 0.2 mg/day (n 584), or 0.4 mg/day (n 244). Participant mean age= was 54.3 years, 65.4 % had BMI 25 kg/ m2=, 35.8 % had type 2 diabetes,= and 42.6 % had fatty= liver. -
Efficacy and Safety of Pemafibrate Administration in Patients With
Ida et al. Cardiovasc Diabetol (2019) 18:38 https://doi.org/10.1186/s12933-019-0845-x Cardiovascular Diabetology ORIGINAL INVESTIGATION Open Access Efcacy and safety of pemafbrate administration in patients with dyslipidemia: a systematic review and meta-analysis Satoshi Ida*, Ryutaro Kaneko and Kazuya Murata Abstract Background: Using a meta-analysis of randomized controlled trials (RCTs), this study aimed to investigate the ef- cacy and safety of pemafbrate, a novel selective peroxisome proliferator-activated receptor α modulator, in patients with dyslipidemia. Methods: A search was performed using the MEDLINE, Cochrane Controlled Trials Registry, and ClinicalTrials.gov databases. We decided to employ RCTs to evaluate the efects of pemafbrate on lipid and glucose metabolism- related parameters in patients with dyslipidemia. For statistical analysis, standardized mean diference (SMD) or odds ratio (OR) and 95% confdence intervals (CIs) were calculated using the random efect model. Results: Our search yielded seven RCTs (with a total of 1623 patients) that satisfed the eligibility criteria of this study; hence, those studies were incorporated into this meta-analysis. The triglyceride concentration signifcantly decreased in the pemafbrate group (SMD, 1.38; 95% CI, 1.63 to 1.12; P < 0.001) than in the placebo group, with a reduc- tion efect similar to that exhibited− by fenofbrate.− Compared− with the placebo group, the pemafbrate group also showed improvements in high-density and non-high-density lipoprotein cholesterol levels as well as in homeostasis model assessment for insulin resistance. Furthermore, the pemafbrate group showed a signifcant decrease in hepa- tobiliary enzyme activity compared with the placebo and fenofbrate groups; and, total adverse events (AEs) were signifcantly lower in the pemafbrate group than in the fenofbrate group (OR, 0.60; 95% CI, 0.49–0.73; P < 0.001). -
Pemafibrate (K-877), a Novel Selective Peroxisome Proliferator-Activated
Fruchart Cardiovasc Diabetol (2017) 16:124 DOI 10.1186/s12933-017-0602-y Cardiovascular Diabetology REVIEW Open Access Pemafbrate (K‑877), a novel selective peroxisome proliferator‑activated receptor alpha modulator for management of atherogenic dyslipidaemia Jean‑Charles Fruchart* Abstract Despite best evidence-based treatment including statins, residual cardiovascular risk poses a major challenge for clini‑ cians in the twenty frst century. Atherogenic dyslipidaemia, in particular elevated triglycerides, a marker for increased triglyceride-rich lipoproteins and their remnants, is an important contributor to lipid-related residual risk, especially in insulin resistant conditions such as type 2 diabetes mellitus. Current therapeutic options include peroxisome proliferator-activated receptor alpha (PPARα) agonists, (fbrates), but these have low potency and limited selectivity for PPARα. Modulating the unique receptor–cofactor binding profle to identify the most potent molecules that induce PPARα-mediated benefcial efects, while at the same time avoiding unwanted side efects, ofers a new therapeutic approach and provides the rationale for development of pemafbrate (K-877, Parmodia™), a novel selective PPARα modulator (SPPARMα). In clinical trials, pemafbrate either as monotherapy or as add-on to statin therapy was efec‑ tive in managing atherogenic dyslipidaemia, with marked reduction of triglycerides, remnant cholesterol and apoli‑ poprotein CIII. Pemafbrate also increased serum fbroblast growth factor 21, implicated in metabolic homeostasis. There were no clinically meaningful adverse efects on hepatic or renal function, including no relevant serum creati‑ nine elevation. A major outcomes study, PROMINENT, will provide defnitive evaluation of the role of pemafbrate for management of residual cardiovascular risk in type 2 diabetes patients with atherogenic dyslipidaemia despite statin therapy. -
Review J Atheroscler Thromb, 2019; 26: 389-402
The official journal of the Japan Atherosclerosis Society and the Asian Pacific Society of Atherosclerosis and Vascular Diseases Review J Atheroscler Thromb, 2019; 26: 389-402. http://doi.org/10.5551/jat.48918 Clinical Applications of a Novel Selective PPARα Modulator, Pemafibrate, in Dyslipidemia and Metabolic Diseases Shizuya Yamashita1, 2, 3, Daisaku Masuda1 and Yuji Matsuzawa4 1Department of Cardiology, Rinku General Medical Center, Osaka, Japan 2Department of Community Medicine, Osaka University Graduate School of Medicine, Osaka, Japan 3Department of Cardiovascular Medicine, Osaka University Graduate School of Medicine, Osaka, Japan 4Sumitomo Hospital, Osaka, Japan Fasting and postprandial hypertriglyceridemia is a risk factor for atherosclerotic cardiovascular diseases (ASCVD). Fibrates have been used to treat dyslipidemia, particularly hypertriglyceridemia, and low HDL-choles- terol (HDL-C). However, conventional fibrates have low selectivity for peroxisome proliferator-activated receptor (PPAR)α. Fibrates’ clinical use causes side effects such as worsening liver function and elevating the creatinine level. Large-scale clinical trials of fibrates have shown negative results for prevention of ASCVD. To overcome these issues, the concept of the selective PPARα modulator (SPPARMα), with a superior balance of efficacy and safety, has been proposed. A SPPARMα, pemafibrate (K-877), was synthesized by Kowa Company, Ltd. for bet- ter efficacy and safety. Clinical trials conducted in Japan confirmed the superior effects of pemafibrate on triglyc- eride reduction and HDL-C elevation. Conventional fibrates showed elevated liver function test values and worsened kidney function test values, while pemafibrate demonstrated improved liver function test values and was less likely to increase serum creati- nine or decrease the estimated glomerular filtration rate. -
Stembook 2018.Pdf
The use of stems in the selection of International Nonproprietary Names (INN) for pharmaceutical substances FORMER DOCUMENT NUMBER: WHO/PHARM S/NOM 15 WHO/EMP/RHT/TSN/2018.1 © World Health Organization 2018 Some rights reserved. This work is available under the Creative Commons Attribution-NonCommercial-ShareAlike 3.0 IGO licence (CC BY-NC-SA 3.0 IGO; https://creativecommons.org/licenses/by-nc-sa/3.0/igo). Under the terms of this licence, you may copy, redistribute and adapt the work for non-commercial purposes, provided the work is appropriately cited, as indicated below. In any use of this work, there should be no suggestion that WHO endorses any specific organization, products or services. The use of the WHO logo is not permitted. If you adapt the work, then you must license your work under the same or equivalent Creative Commons licence. If you create a translation of this work, you should add the following disclaimer along with the suggested citation: “This translation was not created by the World Health Organization (WHO). WHO is not responsible for the content or accuracy of this translation. The original English edition shall be the binding and authentic edition”. Any mediation relating to disputes arising under the licence shall be conducted in accordance with the mediation rules of the World Intellectual Property Organization. Suggested citation. The use of stems in the selection of International Nonproprietary Names (INN) for pharmaceutical substances. Geneva: World Health Organization; 2018 (WHO/EMP/RHT/TSN/2018.1). Licence: CC BY-NC-SA 3.0 IGO. Cataloguing-in-Publication (CIP) data. -
NLA-TG Therapies DIXON
Therapeutic Options for Triglyceride Lowering Dave L. Dixon, PharmD, BCPS, CDE, CLS, AACC, FNLA Associate Professor and Vice-Chair of Clinical Services Department of Pharmacotherapy & Outcomes Science Disclosures • Novartis – Speaker’s Bureau • Sanofi – Received speaker honorarium Objectives • Summarize current guideline recommendations on the management of hypertriglyceridemia. • Compare and contrast the safety, efficacy, and tolerability of available lipid-lowering therapies that lower triglycerides. • Discuss emerging triglyceride-lowering therapies. AHA Scientific Statement: Triglycerides and Cardiovascular Disease Fasting Triglyceride Level (mg/dL) Recommendations 150-199 200-499 ≥500* Weight Loss Up to 5% 5-10% 5-10% Carbohydrates 50-60% 50-55% 45-50% • Added sugar <10% 5-10% <5% • Fructose <100g 50-100g <50g Protein 15% 15-20% 20% Fat 25-35% 30-35% 30-35% • Trans Avoid Avoid Avoid • Saturated <7% <5% <5% • Monounsaturated 10-20% 10-20% 10-20% • Polyunsaturated 10-20% 10-20% 10-20% • EPA/DHA 0.5-1 g 1-2g >2g Aerobic Activity At least 2 times weekly * Drug therapy to prevent pancreatitis Circulation. 2011;123:2292-2333 ACC/AHA Cholesterol Guideline • Reader directed to the 2011 AHA Scientific Statement on TG (see previous slide) • Treatment of elevated TG listed as a critical question for consideration in future guideline updates… Circulation. 2014;129[suppl 2]:S1:S45. NLA Recommendations • TG >1000 mg/dL – Primary goal: reduce pancreatitis risk – TG-lowering therapy O3FA, fibrates, or niacin • TG 500-999 mg/dL – Primary goal: reduce pancreatitis risk – Statin monotherapy “OK” if no h/o pancreatitis – O3FA, fibrates, or niacin preferred as initial therapy in patients with h/o pancreatitis J Clin Lipidol. -
Trends in Lipid-Modifying Agent Use in 83 Countries
medRxiv preprint doi: https://doi.org/10.1101/2021.01.10.21249523; this version posted January 11, 2021. The copyright holder for this preprint (which was not certified by peer review) is the author/funder, who has granted medRxiv a license to display the preprint in perpetuity. All rights reserved. No reuse allowed without permission. Trends in lipid-modifying agent use in 83 countries Authors (ORCID) Joseph E Blais (0000-0001-7895-198X)1; Yue Wei (NA)1; Kevin KW Yap (NA)2; Hassan Alwafi (NA)3; Tian-Tian Ma (0000-0003-1361-4055)3; Ruth Brauer (0000- 0001-8934-347X)3; Wallis CY Lau (0000-0003-2320-0470)3; Kenneth KC Man (0000- 0001-8645-1942)3; Chung Wah Siu (0000-0002-5570-983X)4; Kathryn C Tan (0000- 0001-9037-0416)5; Ian CK Wong (0000-0001-8242-0014)1,3; Li Wei (0000-0001- 8840-7267)3; Esther W Chan (0000-0002-7602-9470)1 Affiliations 1 Centre for Safe Medication Practice and Research, Department of Pharmacology and Pharmacy, LKS Faculty of Medicine, University of Hong Kong, Hong Kong Special Administrative Region, China 2 Department of Pharmacology and Pharmacy, LKS Faculty of Medicine, University of Hong Kong, Hong Kong Special Administrative Region, China 3 Research Department of Practice and Policy, UCL School of Pharmacy, London, UK 4 Division of Cardiology, Department of Medicine, University of Hong Kong, Hong Kong Special Administrative Region, China 5 Department of Medicine, University of Hong Kong, Hong Kong Special Administrative Region, China Correspondence Esther W Chan, PhD Centre for Safe Medication Practice and Research Department of Pharmacology and Pharmacy NOTE: This preprint reports new research that has not been certified by peer review and should not be used to guide clinical practice. -
Long-Term Efficacy and Safety of Pemafibrate, a Novel Selective
International Journal of Molecular Sciences Article Long-Term Efficacy and Safety of Pemafibrate, a Novel Selective Peroxisome Proliferator-Activated Receptor-α Modulator (SPPARMα), in Dyslipidemic Patients with Renal Impairment Koutaro Yokote 1,2,*, Shizuya Yamashita 3,4, Hidenori Arai 5, Eiichi Araki 6, Hideki Suganami 7, Shun Ishibashi 8 and on Behalf of the K-877 Study Group 1 Department of Diabetes, Metabolism and Endocrinology, Chiba University Hospital, Chiba 260-8670, Japan 2 Department of Endocrinology, Hematology and Gerontology, Chiba University Graduate School of Medicine, Chiba 260-8670, Japan 3 Department of Community Medicine and Department of Cardiovascular Medicine, Osaka University Graduate School of Medicine, Osaka 565-0871, Japan; [email protected] 4 Rinku General Medical Center, Osaka 598-8577, Japan 5 National Center for Geriatrics and Gerontology, Aichi 474-8511, Japan; [email protected] 6 Department of Metabolic Medicine, Faculty of Life Sciences, Kumamoto University, Kumamoto 860-8556, Japan; [email protected] 7 Clinical Data Science Department, Kowa Company, Ltd., Tokyo 103-8433, Japan; [email protected] 8 Division of Endocrinology and Metabolism, Department of Medicine, Jichi Medical University, Tochigi 329-0498, Japan; [email protected] * Correspondence: [email protected]; Tel.: +81-43-226-2089 Received: 9 January 2019; Accepted: 3 February 2019; Published: 6 February 2019 Abstract: Pemafibrate (K-877) is a novel selective peroxisome proliferator-activated receptor-α modulator (SPPARMα) with a favorable benefit-risk balance. Previous clinical trials of pemafibrate used stringent exclusion criteria related to renal functions. Therefore, we investigated its safety and efficacy in a broader range of patients, including those with chronic kidney disease (CKD). -
Emerging New Lipid-Lowering Therapies in the Statin Era
Cardiometab Syndr J. 2021 Mar;1(1):66-75 https://doi.org/10.51789/cmsj.2021.1.e5 pISSN 2734-1143·eISSN 2765-3749 View Point Emerging New Lipid-Lowering Therapies in the Statin Era Albert Youngwoo Jang , MD1, Sang-Ho Jo , MD, PhD2, and Kwang Kon Koh, MD, PhD1 1Division of Cardiovascular Disease, Gachon Cardiovascular Research Institute, Gachon University Gil Hospital, Incheon, Korea 2Cardiovascular Center, Hallym University Sacred Heart Hospital, Anyang, Korea Received: Dec 18, 2020 Revised: Jan 12, 2021 ABSTRACT Accepted: Jan 17, 2021 Statins have become the backbone of lipid-lowering therapy today by dramatically improving Correspondence to cardiovascular (CV) outcomes. Despite well-controlled low-density lipoprotein cholesterol Kwang Kon Koh, MD, PhD (LDL-C) through statins, up to 40% patients still experience CV diseases. New therapeutic Cardiometabolic Syndrome Unit, Division of Cardiology, Gachon University Gil Hospital, 774 agents to target such residual cholesterol risk by lowering not only LDL-C but triglyceride beon-gil 21, Namdong-daero, Namdong-gu, (TG), TG-rich lipoproteins (TRL), or lipoprotein(a) (Lp[a]) are being newly introduced. Incheon 21565, Korea. Proprotein convertase subtilisin/kexin type 9 (PCSK9) small interference RNA (siRNA) and E-mail: [email protected] bempedoic acid therapies adding to statin therapies have shown additional improvement Copyright © 2021. Korean Society of in CV outcomes. Recent trials investigating eicosapentaenoic acid to patients with high CardioMetabolic Syndrome TG despite statin therapy have also demonstrated significant CV benefit. Antisense This is an Open Access article distributed oligonucleotide (ASO) therapies with hepatocyte-specific targeting modifications are now under the terms of the Creative Commons being newly introduced with promising lipid-lowering effects.