Pap1 Is an Extrcopy Suppressor of Rad26:4A, a Mutation That Inhibits the Interphase Microtubule Damage Checkpoint of S
Total Page:16
File Type:pdf, Size:1020Kb
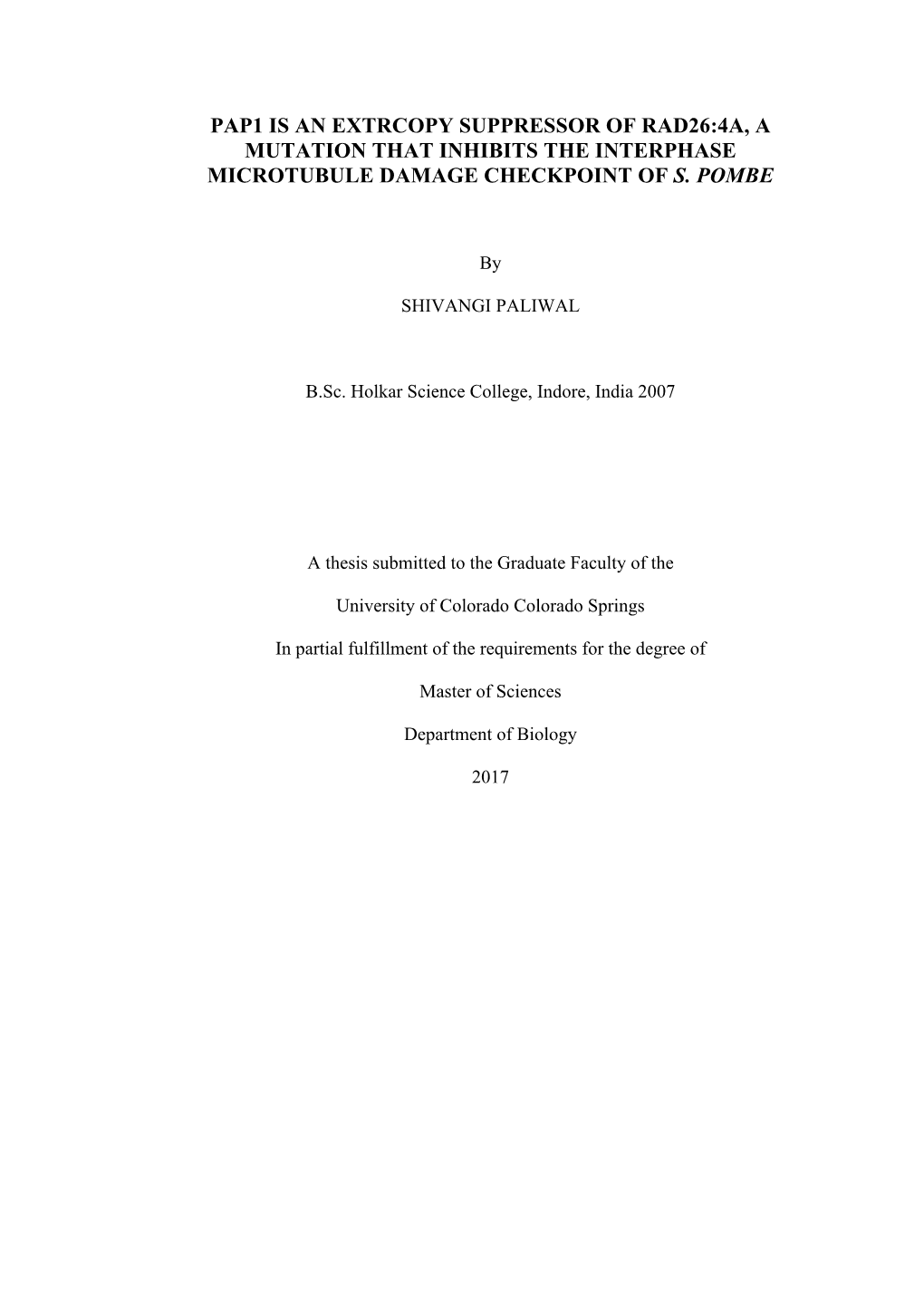
Load more
Recommended publications
-
FAK Nuclear Export Signal Sequences
FEBS Letters 582 (2008) 2402–2406 FAK nuclear export signal sequences Valeria Ossovskayaa,1, Ssang-Taek Limb, Nobuyuki Otac, David D. Schlaepferb, Dusko Ilicc,d,* a Department of Anatomy, University of California San Francisco, San Francisco, CA, USA b Department of Reproductive Medicine, University of California, San Diego, CA, USA c A-cube Inc., Burlingame, CA, USA d Department of Cell and Tissue Biology, University of California San Francisco, San Francisco, CA, USA Received 13 April 2008; revised 28 May 2008; accepted 1 June 2008 Available online 10 June 2008 Edited by Varda Rotter accumulation. R177/R178A mutations also prevented FERM Abstract Ubiquitously expressed focal adhesion kinase (FAK), a critical component in transducing signals from sites of cell con- nuclear localization [12]. tacts with extracellular matrix, was named after its typical local- Since it is found in both cytoplasm and nucleus, FAK obvi- ization in focal adhesions. A nuclear localization of FAK has ously has to have a mechanism that enables nucleocytoplasmic been also reported and its scaffolding role in nucleus and require- shuttling. Leucine-rich nuclear export signal (NES) sequences ment for p53 ubiquitination were only recently described. often mediate protein export from the nucleus to the cyto- Whereas FAK nuclear localization signal (NLS) was found in plasm [13–16]. The first NES were identified in human immu- F2 lobe of FERM domain, nuclear export signal (NES) nodeficiency virus, type I-coded Rev protein [17] and protein sequences have not been yet determined. Here we demonstrate kinase A inhibitor of cAMP-dependent protein kinase [18]. that FAK has two NES sequences, NES1 in F1 lobe of FERM NES sequences consist of 4–5 hydrophobic residues within a domain and NES2 in kinase domain. -
Bioinformatic Analysis of Structure and Function of LIM Domains of Human Zyxin Family Proteins
International Journal of Molecular Sciences Article Bioinformatic Analysis of Structure and Function of LIM Domains of Human Zyxin Family Proteins M. Quadir Siddiqui 1,† , Maulik D. Badmalia 1,† and Trushar R. Patel 1,2,3,* 1 Alberta RNA Research and Training Institute, Department of Chemistry and Biochemistry, University of Lethbridge, 4401 University Drive, Lethbridge, AB T1K 3M4, Canada; [email protected] (M.Q.S.); [email protected] (M.D.B.) 2 Department of Microbiology, Immunology and Infectious Disease, Cumming School of Medicine, University of Calgary, 3330 Hospital Drive, Calgary, AB T2N 4N1, Canada 3 Li Ka Shing Institute of Virology, University of Alberta, Edmonton, AB T6G 2E1, Canada * Correspondence: [email protected] † These authors contributed equally to the work. Abstract: Members of the human Zyxin family are LIM domain-containing proteins that perform critical cellular functions and are indispensable for cellular integrity. Despite their importance, not much is known about their structure, functions, interactions and dynamics. To provide insights into these, we used a set of in-silico tools and databases and analyzed their amino acid sequence, phylogeny, post-translational modifications, structure-dynamics, molecular interactions, and func- tions. Our analysis revealed that zyxin members are ohnologs. Presence of a conserved nuclear export signal composed of LxxLxL/LxxxLxL consensus sequence, as well as a possible nuclear localization signal, suggesting that Zyxin family members may have nuclear and cytoplasmic roles. The molecular modeling and structural analysis indicated that Zyxin family LIM domains share Citation: Siddiqui, M.Q.; Badmalia, similarities with transcriptional regulators and have positively charged electrostatic patches, which M.D.; Patel, T.R. -
Nucleocytoplasmic Transport in Apoptosis
Nucleocytoplasmic transport in apoptosis E Ferrando-May*,1 Introduction 1 Molecular Toxicology Group, Faculty of Biology, University of Konstanz, PO The separation of the nucleus and the cytoplasm is the Box X911, 78457 Konstanz, Germany defining feature of eukaryotic cells and is achieved by the * Corresponding author: E Ferrando-May; Tel: þ 49 7531 884054; nuclear envelope, a double-membrane system of highly Fax: þ 49 7531 884033; E-mail: [email protected] selective permeability. Interchange of material between these two compartments occurs through dedicated transport chan- nels perforating the nuclear envelope, the nuclear pore complexes (NPCs). These are elaborate supramolecular structures consisting of about 30 different proteins, most of Abstract which are termed nucleoporins (Nups). The composition and structure of the NPC have been analysed in detail by a The apoptotic demolition of the nucleus is accomplished by combination of proteomics and electron microscopy ap- diverse proapoptotic factors, most of which are activated in proaches both in yeast and vertebrates, leading to a refined the cytoplasm and gain access to the nucleoplasm during the view of its molecular architecture. Essentially, the NPC is cell death process. The nucleus is also the main target for composed of three substructures of eight-fold rotational genotoxic insult, a potent apoptotic trigger. Signals generated symmetry: the cytoplasmic fibrils, the central framework, in the nucleus by DNA damage have to propagate to all and the nuclear basket (Figure 1). In the central framework, cellular compartments to ensure the coordinated execution of Nups form distinct subcomplexes which are arranged cell demise. The nucleocytoplasmic shuttling of signalling symmetrically with respect to the plane of the nuclear and execution factors is thus an integral part of the apoptotic envelope and enclose the central pore channel. -
Multiple Roles of Phosphoinositide-Specific Phospholipase C Isozymes
BMB reports Mini Review Multiple roles of phosphoinositide-specific phospholipase C isozymes Pann-Ghill Suh1,*, Jae-Il Park1, Lucia Manzoli2, Lucio Cocco2, Joanna C. Peak3, Matilda Katan3, Kiyoko Fukami4, Tohru Kataoka5, Sanguk Yun1 & Sung Ho Ryu1 1Division of Molecular and Life Sciences, Pohang University of Science and Technology, Pohang, Korea, 2Cellular Signaling Laboratory, Department of Anatomical Sciences, University of Bologna, Via Irnerio, 48 I-40126, Bologna, Italy, 3Cancer Research UK Centre for Cell and Molecular Biology, Chester Beatty Laboratories, The Institute of Cancer Research, Fulham Road, London SW3 6JB, UK, 4Laboratory of Genome and Biosignal, Tokyo University of Pharmacy and Life Science, 1432-1 Horinouchi, Hachioji, 192-0392 Tokyo, Japan, 5Division of Molecular Biology, Department of Biochemistry and Molecular Biology, Kobe University Graduate School of Medicine, Chuo-ku, Kobe, Japan Phosphoinositide-specific phospholipase C is an effector mole- cellular calcium release (1-3; Fig. 1a). cule in the signal transduction process. It generates two sec- The first evidence of PLC activity was suggested by Hokin et ond messengers, inositol-1,4,5-trisphosphate and diacylglycer- al. in 1953 who reported specific hydrolysis of phospholipids ol from phosphatidylinositol 4,5-bisphosphate. Currently, thir- in pigeon’s pancreas slices after cholinergic stimulation (4). teen mammal PLC isozymes have been identified, and they are The authors showed that the enhanced turnover of phosphor- divided into six groups: PLC-β, -γ, -δ, -ε, -ζ and -η. Sequence ylinositol groups of phosphatidylinositol occurred in cells as a analysis studies demonstrated that each isozyme has more response to a variety of stimuli. In 1983, Streb et al. -
Exploring Machine Learning Methods for Nuclear Export Sequence Identification
Exploring Machine Learning Methods for Nuclear Export Sequence Identification A Major Qualifying Project Report Submitted to the Faculty Of the WORCESTER POLYTECHNIC INSTITUTE In partial fulfillment of the requirements for the Degree of Bachelor of Science ______________________________ Erin Conneilly Advisors: ______________________________ Professor Destin Heilman (CBC) ______________________________ Professor Rodica Neamtu (CS) Abstract The goal of this project is to design and implement a user-friendly machine learning tool that can be applied to the classification of polypeptides to find functional nuclear export sequences (NESs). This tool incorporates an API that takes advantage of support vector machines and can be expanded to include other models. Because NESs have been found to have consistent structure, structural data is incorporated into the model to increase confidence. This report is accompanied by a manual that instructs users on how to use the tool. 1 Table of Contents Abstract 1 Table of Contents 2 Table of Figures 3 Table of Tables 3 Acknowledgements 4 Introduction 5 Project Motivation 5 Problem Statement 5 Background 6 Section 1: Intracellular Transport 6 Section 2: Nuclear Export Sequence Structure 8 Section 3: Existing Prediction Methods 9 Section 4: Machine Learning Techniques 9 4.1 Recurrent Neural Networks (RNNs) 10 4.2 Nuclear Export Sequences as Time Series 10 4.3 Autoregressive Integrated Moving Average Model (ARIMA) 11 4.4 One-Class Support Vector Machines (OC SVM) 11 4.5 Scikit-Learn 13 Tool Overview and -
Subcellular Localization of Crma: Identification of a Novel Leucine-Rich
Biochem. J. (2003) 373, 251–259 (Printed in Great Britain) 251 Subcellular localization of CrmA: identification of a novel leucine-rich nuclear export signal conserved in anti-apoptotic serpins Jose A. RODRIGUEZ1,Simone W. SPAN, Frank A. E. KRUYT and Giuseppe GIACCONE Department of Medical Oncology, VU University Medical Center, HV1081 Amsterdam, The Netherlands The cowpox virus-encoded anti-apoptotic protein cytokine flanking the NES contribute to the CRM1-dependent nuclear response modifier A (CrmA) is a member of the serpin family export of CrmA. Although YFP-tagged CrmA is primarily located that specifically inhibits the cellular proteins caspase 1, caspase in the cytoplasm, shifting its localization to be predominantly 8andgranzyme B. In this study, we have used Flag- and yellow nuclear by fusion of a heterologous nuclear localization signal fluorescent protein (YFP)-tagged versions of CrmA to investigate did not impair its ability to prevent Fas-induced apoptosis. We the mechanisms that regulate its subcellular localization. We show propose that nucleocytoplasmic shuttling would allow CrmA to that CrmA can actively enter and exit the nucleus and we dem- efficiently target cellular pro-apoptotic proteins not only in the onstrate the role of the nuclear export receptor CRM1 in this cytoplasm, but also in the nucleus, and thus to carry out its anti- shuttling process. CrmA contains a novel leucine-rich nuclear apoptotic function in both compartments. export signal (NES) that is functionally conserved in the anti- apoptotic cellular serpin PI-9. Besides this leucine-rich export signal, additional sequences mapping to a 103-amino-acid region Keywords: apoptosis, nuclear transport, CRM1. -
Active Maintenance of Nuclear Actin by Importin 9 Supports Transcription
Active maintenance of nuclear actin by PNAS PLUS importin 9 supports transcription Joseph Dopiea,1, Kari-Pekka Skarpa,1, Eeva Kaisa Rajakyläa, Kimmo Tanhuanpääb, and Maria K. Vartiainena,2 aProgram in Cell and Molecular Biology and bLight Microscopy Unit, Institute of Biotechnology, University of Helsinki, 00014 Helsinki, Finland Edited by Larry Gerace, The Scripps Research Institute, La Jolla, CA, and accepted by the Editorial Board January 4, 2012 (received for review November 17, 2011) Besides its essential and well established role as a component of that these biochemical properties of actin are utilized also within the cytoskeleton, actin is also present in the cell nucleus, where the nucleus. Indeed, actin and nuclear myosins have been impli- it has been linked to many processes that control gene expression. cated in the movement of individual gene loci upon transcription For example, nuclear actin regulates the activity of specific tran- activation (14–16) and even in rearranging whole chromosomes scription factors, associates with all three RNA polymerases, and is within the nucleus (17). Furthermore, although the functional con- a component of many chromatin remodelling complexes. Despite formation of nuclear actin is still under debate (18, 19), many of the the fact that two export receptors, Crm1 and exportin 6, have been nuclear actin related functions mentioned above are disrupted linked to nuclear export of actin, the mechanism by which actin upon treatment of cells with drugs affecting actin polymerization enters the nucleus to elicit these essential functions has not been (15, 20, 21), suggesting that actin polymerization plays a role also determined. It is also unclear whether actin is actively exchanged in the cell nucleus. -
Cytoplasmic Role in Actin Polymerization Is Uncoupled From
Nuclear Role of WASp in Gene Transcription Is Uncoupled from Its ARP2/3-Dependent Cytoplasmic Role in Actin Polymerization This information is current as Sanjoy Sadhukhan, Koustav Sarkar, Matthew Taylor, Fabio of September 25, 2021. Candotti and Yatin M. Vyas J Immunol 2014; 193:150-160; Prepublished online 28 May 2014; doi: 10.4049/jimmunol.1302923 http://www.jimmunol.org/content/193/1/150 Downloaded from Supplementary http://www.jimmunol.org/content/suppl/2014/05/27/jimmunol.130292 Material 3.DCSupplemental http://www.jimmunol.org/ References This article cites 51 articles, 24 of which you can access for free at: http://www.jimmunol.org/content/193/1/150.full#ref-list-1 Why The JI? Submit online. • Rapid Reviews! 30 days* from submission to initial decision by guest on September 25, 2021 • No Triage! Every submission reviewed by practicing scientists • Fast Publication! 4 weeks from acceptance to publication *average Subscription Information about subscribing to The Journal of Immunology is online at: http://jimmunol.org/subscription Permissions Submit copyright permission requests at: http://www.aai.org/About/Publications/JI/copyright.html Email Alerts Receive free email-alerts when new articles cite this article. Sign up at: http://jimmunol.org/alerts The Journal of Immunology is published twice each month by The American Association of Immunologists, Inc., 1451 Rockville Pike, Suite 650, Rockville, MD 20852 All rights reserved. Print ISSN: 0022-1767 Online ISSN: 1550-6606. The Journal of Immunology Nuclear Role of WASp in Gene Transcription Is Uncoupled from Its ARP2/3-Dependent Cytoplasmic Role in Actin Polymerization Sanjoy Sadhukhan,*,1 Koustav Sarkar,*,†,1 Matthew Taylor,* Fabio Candotti,‡ and Yatin M. -
An Update on Nucleocytoplasmic Transport in Apoptosis
Commuting (to) suicide: An update on nucleocytoplasmic transport in apoptosis Patricia Grote, Karin Schaeuble, Elisa Ferrando-May" Ulliversity of KOllstanz, Departmellt of Biology, Molecular Toxicology, P.O. Box X911, D 78457 KOllstall z, GermallY Abstract Commuting is the process of travelling between a place of residence and a place of work. In the context of biology, this expression evokes the continuous movement of macromolecules between different compartments of a eukaryotic cell. Transport in and out of the nucleus is a major example of intracellular commuting. This article discusses recent findings that substantiate the emerging link between nucleocytoplasmic transport and the signalling and execution of cell death. KeYlVords: Caspase; Nuclear pore; DNA damage; Nuclear per~eability; Cellular stress; Ran; NFKB; GAPDH; PIDD; Virus Interorganellar cross-talk has become a fundamental of the nuclear pore complex (NPC) I in dying cells, (2) issue in the field of cell death by apoptosis. Distinct cel mechanisms of nuclear uptake/release of individual apo lular organelles and compartments such as the mitochon ptosis mediators, and (3) stress-induced changes in global dria, the endoplasmic reticulum, the nucleus and the transport activity. The present mini review highlights plasma membrane can be the source of primary signals some recent results related to each of these three topics. that lead to cell killing [1,2]. Conversely, downstream For a more detailed introduction into the molecular effectors of cell death, primarily caspases, act at different biology of the NPC and the mechanisms of nuclear subcellular sites to accomplish the dismantling of cellular transport, several excellent review articles are available structures. -
The Role of Protein Disorder in Nuclear Transport and in Its Subversion by Viruses
cells Review The Role of Protein Disorder in Nuclear Transport and in Its Subversion by Viruses Jacinta M. Wubben 1,2, Sarah C. Atkinson 1,2 and Natalie A. Borg 1,2,* 1 Chronic Infectious and Inflammatory Diseases Research, School of Health and Biomedical Sciences, RMIT University, Bundoora, VIC 3083, Australia; [email protected] (J.M.W.); [email protected] (S.C.A.) 2 Infection and Immunity Program, Monash Biomedicine Discovery Institute and Department of Biochemistry and Molecular Biology, Monash University, Clayton, VIC 3800, Australia * Correspondence: [email protected] Received: 12 October 2020; Accepted: 8 December 2020; Published: 10 December 2020 Abstract: The transport of host proteins into and out of the nucleus is key to host function. However, nuclear transport is restricted by nuclear pores that perforate the nuclear envelope. Protein intrinsic disorder is an inherent feature of this selective transport barrier and is also a feature of the nuclear transport receptors that facilitate the active nuclear transport of cargo, and the nuclear transport signals on the cargo itself. Furthermore, intrinsic disorder is an inherent feature of viral proteins and viral strategies to disrupt host nucleocytoplasmic transport to benefit their replication. In this review, we highlight the role that intrinsic disorder plays in the nuclear transport of host and viral proteins. We also describe viral subversion mechanisms of the host nuclear transport machinery in which intrinsic disorder is a feature. Finally, we discuss nuclear import and export as therapeutic targets for viral infectious disease. Keywords: protein intrinsic disorder; nuclear transport receptors; nuclear export sequence; nuclear import sequence; nucleoporins; viral infection; nuclear import inhibitors; nuclear export inhibitors 1. -
A Nuclear Export Signal Is Essential for the Cytosolic Localization of the Ran Binding Protein, Ranbp1 Stephanie A
A Nuclear Export Signal Is Essential for the Cytosolic Localization of the Ran Binding Protein, RanBP1 Stephanie A. Richards, Karen M. Lounsbury, Kimberly L. Carey, and Ian G. Macara Departments of Pathology and Microbiology/Molecular Genetics, University of Vermont, Burlington, Vermont 05405-0068 Abstract. RanBP1 is a Ran/TC4 binding protein that contains a nuclear export signal that is necessary but can promote the interaction between Ran and 13-impor- not sufficient for the nuclear export of a functional tin/13-karyopherin, a component of the docking com- RBD. In transiently transfected cells, epitope-tagged plex for nuclear protein cargo. This interaction occurs RanBP1 promotes dexamethasone-dependent nuclear through a Ran binding domain (RBD). Here we show accumulation of a glucocorticoid receptor-green fluo- that RanBP1 is primarily cytoplasmic, but the isolated rescent protein fusion, but the isolated RBD potently RBD accumulates in the nucleus. A region COOH-ter- inhibits this accumulation. The cytosolic location of minal to the RBD is responsible for this cytoplasmic lo- RanBP1 may therefore be important for nuclear pro- calization. This domain acts heterologously, localizing a tein import. RanBP1 may provide a key link between nuclear cyclin B1 mutant to the cytoplasm. The domain the nuclear import and export pathways. RAFFIC between the nucleus and the cytoplasm oc- Rev and hnRNP A1 are involved in the export of RNA curs through nuclear pore complexes in the nuclear from the nucleus (Pifiol-Roma and Dreyfuss, 1992; Fischer T membrane. Proteins containing a nuclear localiza- et al., 1994; for review see Izaurralde and Mattaj, 1995); tion signal (NLS) 1 are actively transported into the nu- PKI is a regulatory subunit of cAMP-dependent protein cleus by the nuclear import machinery. -
NES Consensus Redefined by Structures of PKI-Type and Rev-Type Nuclear Export Signals Bound to CRM1
ARTICLES NES consensus redefined by structures of PKI-type and Rev-type nuclear export signals bound to CRM1 Thomas Güttler1,5, Tobias Madl2,3,5, Piotr Neumann4,5, Danilo Deichsel1, Lorenzo Corsini2,3, Thomas Monecke4, Ralf Ficner4, Michael Sattler2,3 & Dirk Görlich1 Classic nuclear export signals (NESs) confer CRM1-dependent nuclear export. Here we present crystal structures of the RanGTP−CRM1 complex alone and bound to the prototypic PKI or HIV-1 Rev NESs. These NESs differ markedly in the spacing of their key hydrophobic (F) residues, yet CRM1 recognizes them with the same rigid set of five F pockets. The different F spacings are compensated for by different conformations of the bound NESs: in the case of PKI, an a-helical conformation, and in the case of Rev, an extended conformation with a critical proline docking into a F pocket. NMR analyses of CRM1-bound and CRM1-free PKI NES suggest that CRM1 selects NES conformers that pre-exist in solution. Our data lead to a new structure-based NES consensus, and explain why NESs differ in their affinities for CRM1 and why supraphysiological NESs bind the exportin so tightly. Nuclear export is essential for eukaryotic life. It proceeds through HIV-1, for example, uses CRM1 to export its genomic RNA from nuclear pore complexes (NPCs) and is typically accomplished by nuclei4,19,20. The HIV-1 Rev protein is an adaptor in this process. It exportins1,2. The most versatile exportin is CRM1 (also called binds the unspliced viral RNA, recruits CRM1 and thereby triggers exportin 1 or Xpo1p)3–7.