New Worlds Observer Technology Development Plan
Total Page:16
File Type:pdf, Size:1020Kb
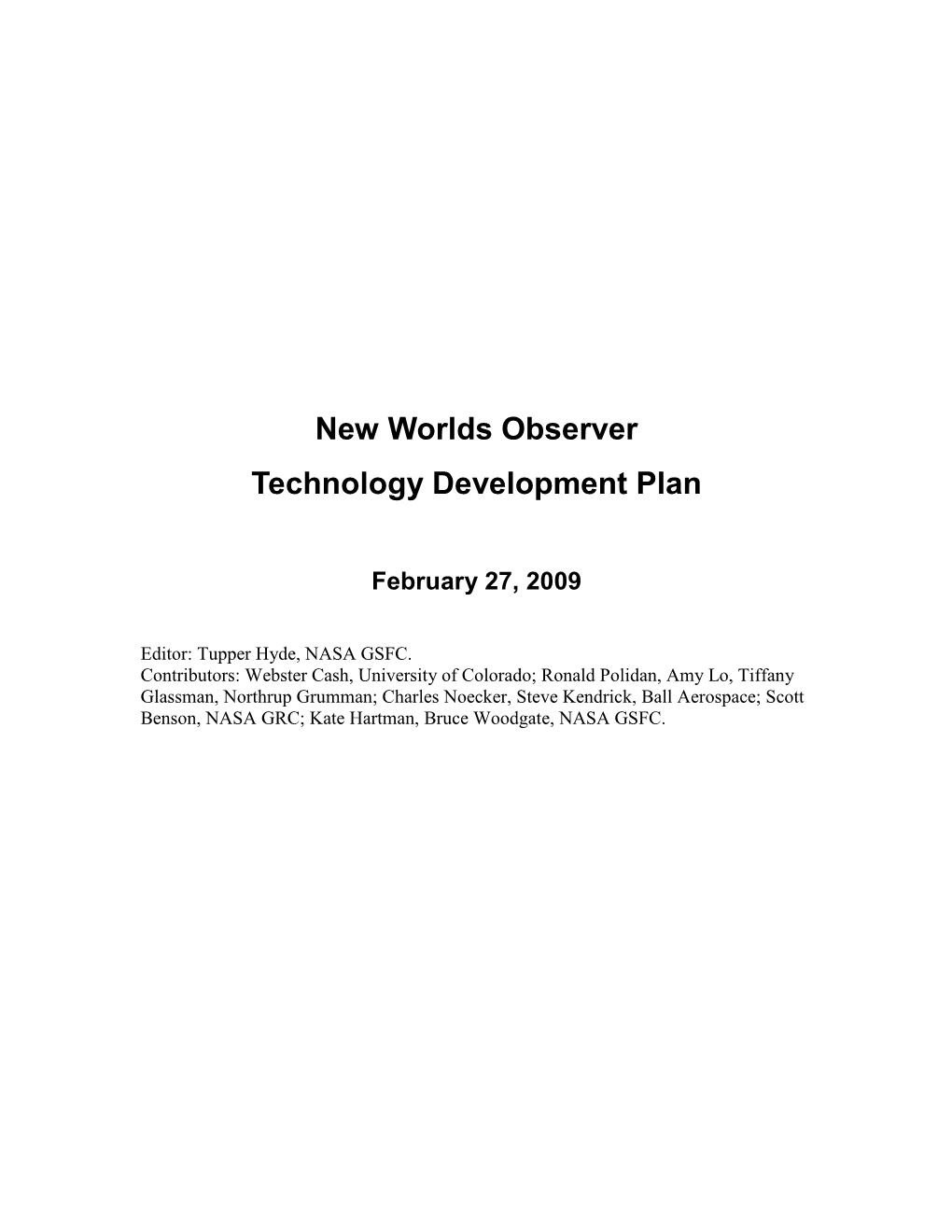
Load more
Recommended publications
-
Astrophysics Division Astrophysics Douglas Hudgins Program Scientist, Exoplanet Exploration Program Key NASA/SMD Science Themes
National Aeronautics and Space Administration NASA and the Search for Life on Planets around Other Stars A presentation to the National Academies Committee on Exoplanet Science Strategy 6 March 2018 Paul Hertz Director, Astrophysics Division Astrophysics Douglas Hudgins Program Scientist, Exoplanet Exploration Program Key NASA/SMD Science Themes Protect and Improve Life on Earth Search for Life Elsewhere Discover the Secrets of the Universe 2 Talk summary 3 NASA’s Exoplanet Exploration Program Space Missions and Mission Studies Public Communications Kepler, WFIRST Decadal Studies K2 Starshade Coronagraph Supporting Research & Technology Key Sustaining Research NASA Exoplanet Science Institute Technology Development Coronagraph Masks Large Binocular Keck Single Aperture Telescope Interferometer Imaging and RV High-Contrast Deployable Archives, Tools, Sagan Fellowships, Imaging Starshades Professional Engagement NN-EXPLORE https://exoplanets.nasa.gov 4 Foundational Documents for the NASA’s Astrophysics Division 5 NASA’s cross-divisional Search for Life Elsewhere ASTROPHYSICS • Exoplanet detection and Planetary SCIENCE/ characterization ASTROBIOLOGY • Stellar characterization • Comparative planetology • Mission data analysis • Planetary atmospheres Hubble, Spitzer, Kepler, • Assessment of observable TESS, JWST, WFIRST, biosignatures etc. • Habitability EARTH SCIENCES • GCM • Planets as systems PLANETARY SCIENCE RESEARCH HELIOPHYSICS • Exoplanet characterization • Stellar characterization • Protoplanetary disks • Stellar winds • Planet formation • Detection of planetary • Comparative planetology magnetospheres 6 Exoplanet Exploration at NASA 2007 - present 7 The Spitzer Space Telescope For the last decade, the Spitzer Space Telescope has used both spectroscopic and photometric measurements in the mid-IR to probe exoplanets and exoplanetary systems. • Spitzer follow up observations of known transiting systems have revealed additional, new planets and helped refine measurements of the size and orbital dynamics of known planets as small as the Earth. -
“Formas Primitivas” De Ideias Oopulares
A FORMA DAS IDEIAS A Câmara Estenopeica Como Método de Destilação de “Formas Primitivas” de Ideias Populares (Expressões Idiomáticas) Salomé Filipa Pereira Arieira Tese de Doutoramento Directora Mª Sol Alonso Romera Facultad de Bellas Artes de Pontevedra Departamento de Dibujo Setembro 2015 Aos meus dois amores: ao meu marido Tony Oliveira e ao meu filho Gaspar, pelo amor infinito e indescritível com que recheiam os meus dias. Aos meus pais Odete e Filipe, pela coragem exemplar e pelo apoio que me transmitem sempre, desde o meu primeiro momento no mundo. À Diretora desta Tese, Sol Alonso, pela disponibilidade total que demonstrou ao longo destes anos para me guiar e acompanhar, e pela amizade e sabedoria com que desde sempre me presenteou. À Direção da Escola Artística de Soares dos Reis por todo o apoio dado ao longo destes últimos anos. Ao Miguel Paiva, à Luisa Fragoso e ao Leonardo Mira, por toda a colaboração e apoio prestados. Ao Miguel Borges (Cigano) pela disponibilidade demonstrada na cedência dos espaços que serviram de palco a várias imagens estenopeicas do projeto prático desta investigação. À Lurdes Arieira e à Claudia Tomás pelo empréstimo de alguns dos elementos fotografados (máquina de picar AGRADECIMENTOS carne e fichas de póquer). À Joana Castelo e ao David, pela gentileza que tiveram na digitalização dos negativos do projeto prático. À Sara e ao André (Blues Photography) pelo cuidado nas impressões fotográficas do projeto prático (versão impressa). À Eduarda Coelho, por me ter salvo no momento da análise sintática das Expressões Idiomáticas. À Maria Peña, cuja amizade se estende desde o início do Curso de Doutoramento, superanto barreiras físicas e temporais. -
Exep Science Plan Appendix (SPA) (This Document)
ExEP Science Plan, Rev A JPL D: 1735632 Release Date: February 15, 2019 Page 1 of 61 Created By: David A. Breda Date Program TDEM System Engineer Exoplanet Exploration Program NASA/Jet Propulsion Laboratory California Institute of Technology Dr. Nick Siegler Date Program Chief Technologist Exoplanet Exploration Program NASA/Jet Propulsion Laboratory California Institute of Technology Concurred By: Dr. Gary Blackwood Date Program Manager Exoplanet Exploration Program NASA/Jet Propulsion Laboratory California Institute of Technology EXOPDr.LANET Douglas Hudgins E XPLORATION PROGRAMDate Program Scientist Exoplanet Exploration Program ScienceScience Plan Mission DirectorateAppendix NASA Headquarters Karl Stapelfeldt, Program Chief Scientist Eric Mamajek, Deputy Program Chief Scientist Exoplanet Exploration Program JPL CL#19-0790 JPL Document No: 1735632 ExEP Science Plan, Rev A JPL D: 1735632 Release Date: February 15, 2019 Page 2 of 61 Approved by: Dr. Gary Blackwood Date Program Manager, Exoplanet Exploration Program Office NASA/Jet Propulsion Laboratory Dr. Douglas Hudgins Date Program Scientist Exoplanet Exploration Program Science Mission Directorate NASA Headquarters Created by: Dr. Karl Stapelfeldt Chief Program Scientist Exoplanet Exploration Program Office NASA/Jet Propulsion Laboratory California Institute of Technology Dr. Eric Mamajek Deputy Program Chief Scientist Exoplanet Exploration Program Office NASA/Jet Propulsion Laboratory California Institute of Technology This research was carried out at the Jet Propulsion Laboratory, California Institute of Technology, under a contract with the National Aeronautics and Space Administration. © 2018 California Institute of Technology. Government sponsorship acknowledged. Exoplanet Exploration Program JPL CL#19-0790 ExEP Science Plan, Rev A JPL D: 1735632 Release Date: February 15, 2019 Page 3 of 61 Table of Contents 1. -
Proxima B: the Alien World Next Door - Is Anyone Home?
Proxima b: The Alien World Next Door - Is Anyone Home? Edward Guinan Biruni Observatory Dept. Astrophysics & Planetary Science th 40 Anniversary Workshop Villanova University 12 October, 2017 [email protected] Talking Points i. Planet Hunting: Exoplanets ii. Living with a Red Dwarf Program iii. Alpha Cen ABC -nearest Star System iv. Proxima Cen – the red dwarf star v. Proxima b Nearest Exoplanet vi. Can it support Life? vii. Planned Observations / Missions Planet Hunting: Finding Exoplanets A brief summary For citizen science projects: www.planethunters.org Early Thoughts on Extrasolar Planets and Life Thousands of years ago, Greek philosophers speculated… “There are infinite worlds both like and unlike this world of ours...We must believe that in all worlds there are living creatures and planets and other things we see in this world.” Epicurius c. 300 B.C First Planet Detected 51 Pegasi – November 1995 Mayer & Queloz / Marcy & Butler Credit: Charbonneau Many Exoplanets (400+) have been detected by the Spectroscopic Doppler Motion Technique (now can measure motions as low as 1 m/s (3.6 km/h = 2.3 mph)) Exoplanet Transit Eclipses Rp/Rs ~ [Depth of Eclipse] 1/2 Transit Eclipse Depths for Jupiter, Neptune and Earth for the Sun 0.01% (Earth-Sun) 0.15% (Neptune-Sun) 1.2% (Jupiter-Sun) Kepler Mission See: kepler.nasa.gov Has so far discovered 6000+ Confirmed & Candidate Exoplanets The Search for Planets Outside Our Solar System Exoplanet Census May 2017 Exoplanet Census (May-2017) Confirmed exoplanets: 3483+ (Doppler / Transit) 490+ Multi-planet Systems [April 2017] Exoplanet Candidates: 7900+ orbiting 2600+ stars (Mostly from the Kepler Mission) [May 2017] Other unconfirmed (mostly from CoRot)Exoplanets ~186+ Potentially Habitable Exoplanets: 51 (April 2017) Estimated Planets in the Galaxy ~ 50 -100 Billion! Most expected to be hosted by red dwarf stars Nomad (Free-floating planets) ~ 25 - 50 Billion Known planets with life: 1 so far. -
The Official Publication of the South African Institute Of
THE OFFICIAL PUBLICATION OF THE SOUTH AFRICAN INSTITUTE OF ELECTRICAL ENGINEERS | OCTOBER 2017 wattnow | october 2017 | 1 Venue Emperors Palace, Ekurhuleni, South Africa Dates 28, 29 and 30 November 2017 Theme Showcasing energy storage technologies and applications as an enabling and disruptive technology. A focussed conference and exhibition covering policy, regulatory, economic, technology, business and application issues associated with Energy Storage, both as a disrupting and an enabling technology. Organised & hosted by 2 | wattnow | Foroctober further 2017 information see website: www.energystorage.co.za 17- SA Energy Storage 2017 - A4 - Wattnow.indd 1 27/09/2017 11:39:59 Venue Emperors Palace, Ekurhuleni, South Africa Dates 28, 29 and 30 November 2017 UNDERGROUND WI-FI COMMS 22 THE LAW CALLS FOR RELIABLE COMMUNICATIONS Theme Showcasing energy storage technologies and applications as an TRIPLE POLAR BAND-PASS FILTER enabling and disruptive technology. FEATURES 36 BANDWIDTH IS BEING OBSERVED FULLY UTILISING IOT IN INDUSTRY 44 COMMUNICATION IS CRUCIAL FOR THE CLOUD 20 MEMBERSHIP NOTIFICATION 47 COMMUNICATING WITH ET 48 HOW FIBRE WILL AIDE SA 56 44 48 WATTSUP 8 WATT? 60 A focussed conference and exhibition covering policy, regulatory, economic, technology, business and LOOKING BACK... OCTOBER application issues associated with Energy Storage, both as a disrupting and an enabling technology. 62 CALENDAR 65 62 Organised & hosted by www.energystorage.co.za For further information see website: SAIEE @saiee wattnow | october 2017 | 3 17- SA Energy Storage 2017 - A4 - Wattnow.indd 1 27/09/2017 11:39:59 MANAGING EDITOR Minx Avrabos | [email protected] TECHNICAL EDITORS Derek Woodburn Jane-Anne Buisson-Street Dear Reader, CONTRIBUTORS We have entered the ‘silly’ season, S Mbanjwa with year-end functions and parties P Motsoasele F Sorkh Abadi filling our diaries! It is because of A Roohavar this that I chose this issue features K Jacobs Communication. -
Mètodes De Detecció I Anàlisi D'exoplanetes
MÈTODES DE DETECCIÓ I ANÀLISI D’EXOPLANETES Rubén Soussé Villa 2n de Batxillerat Tutora: Dolors Romero IES XXV Olimpíada 13/1/2011 Mètodes de detecció i anàlisi d’exoplanetes . Índex - Introducció ............................................................................................. 5 [ Marc Teòric ] 1. L’Univers ............................................................................................... 6 1.1 Les estrelles .................................................................................. 6 1.1.1 Vida de les estrelles .............................................................. 7 1.1.2 Classes espectrals .................................................................9 1.1.3 Magnitud ........................................................................... 9 1.2 Sistemes planetaris: El Sistema Solar .............................................. 10 1.2.1 Formació ......................................................................... 11 1.2.2 Planetes .......................................................................... 13 2. Planetes extrasolars ............................................................................ 19 2.1 Denominació .............................................................................. 19 2.2 Història dels exoplanetes .............................................................. 20 2.3 Mètodes per detectar-los i saber-ne les característiques ..................... 26 2.3.1 Oscil·lació Doppler ........................................................... 27 2.3.2 Trànsits -
OLLI Talk on Exoplanets
OLLI SC211 SPACE EXPLORATION: THE SEARCH FOR LIFE BEYOND EARTH April 13, 2017 Michal Peri NASA Solar System Ambassador Exoplanet2 Detection • Methods • Missions • Discoveries • Resources Image: C. Pulliam & D. Aguilar/CfA A long history … in our imaginations 3 https://en.wikipedia.org/wiki/Giordano_Bruno#/media/File:Relief_Bruno_Campo_dei_Fiori_n1.jpg Giordano Bruno in his De l'infinito universo et mondi (1584) suggested that "stars are other suns with their own planets” that “have no less virtue nor a nature different to that of our earth" and, like Earth, "contain animals and inhabitants.” For this heresy, he was burned by the inquisition. 3 https://www.loc.gov/today/cyberlc/feature_wdesc.php?rec=7148 5 Detection Methods Radial Velocity 619 planets Gravitational Microlensing 44 planets Direct Imaging 44 planets Transit 2771 planets Velocity generates Doppler 7 Shift Sound star receding star approaching Light wave “stretched” → red shift wave “squashed” → blue shift Orbiting planet causes Doppler shift in starlight 8 https://exoplanets.nasa.gov/interactable/11/ Radial Velocity Method 9 • The most successful method of detecting exoplanets pre-2010 • Doppler shifts in the stellar spectrum reveal presence of planetary companion(s) • Measure lower limit of the planetary mass and orbital parameters Doppler Shift Time Nikole K. Lewis, STScI Radial Velocity Detections Radial Velocity 1992 Planetary Mass 10 Detection Methods Radial Velocity 619 planets Gravitational Microlensing 44 planets Direct Imaging 44 planets Transit 2771 planets • Microlens -
Professor Sara Seager Massachusetts Institute of Technology
Professor Sara Seager Massachusetts Institute of Technology Address: Department of Earth Atmospheric and Planetary Science Building 54 Room 1718 Massachusetts Institute of Technology 77 Massachusetts Avenue Cambridge, MA, USA 02139 Phone: (617) 253-6779 (direct) E-mail: [email protected] Citizenship: US citizen since 7/20/2010 Birthdate: 7/21/1971 Professional History 1/2011–present: Massachusetts Institute of Technology, Cambridge, MA USA • Class of 1941 Professor (1/2012–present) • Professor of Planetary Science (7/2010–present) • Professor of Physics (7/2010–present) • Professor of Aeronautical and Astronautical Engineering (7/2017–present) 1/2007–12/2011: Massachusetts Institute of Technology, Cambridge, MA USA • Ellen Swallow Richards Professorship (1/2007–12/2011) • Associate Professor of Planetary Science (1/2007–6/2010) • Associate Professor of Physics (7/2007–6/2010) • Chair of Planetary Group in the Dept. of Earth, Atmospheric, and Planetary Sciences (2007–2015) 08/2002–12/2006: Carnegie Institution of Washington, Washington, DC, USA • Senior Research Staff Member 09/1999–07/2002: Institute for Advanced Study, Princeton NJ • Long Term Member (02/2001–07/2002) • Short Term Member (09/1999–02/2001) • Keck Fellow Educational History 1994–1999 Ph.D. “Extrasolar Planets Under Strong Stellar Irradiation” Department of Astronomy, Harvard University, MA, USA 1990–1994 B.Sc. in Mathematics and Physics University of Toronto, Canada NSERC Science and Technology Fellowship (1990–1994) Awards and Distinctions Academic Awards and Distinctions 2018 American Philosophical Society Member 2018 American Academy of Arts and Sciences Member 2015 Honorary PhD, University of British Columbia 2015 National Academy of Sciences Member 2013 MacArthur Fellow 2012 Raymond and Beverly Sackler Prize in the Physical Sciences 2012 American Association for the Advancement of Science Fellow 2007 Helen B. -
Planets Galore
physicsworld.com Feature: Exoplanets Detlev van Ravenswaay/Science Photo Library Planets galore With almost 1700 planets beyond our solar system having been discovered, climatologists are beginning to sketch out what these alien worlds might look like, as David Appell reports And so you must confess Jupiters, black Jupiters or puffy Jupiters; there are David Appell is a That sky and earth and sun and all that comes to be hot Neptunes and mini-Neptunes; exo-Earths, science writer living Are not unique but rather countless examples of a super-Earths and eyeball Earths. There are planets in Salem, Oregon, class. that orbit pulsars, or dim red dwarf stars, or binary US, www. Lucretius, Roman poet and philosopher, from star systems. davidappell.com De Rerum Natura, Book II Astronomers are in heaven and planetary scien- tists have an entirely new zoo to explore. “This is the The only thing more astonishing than their diver- best time to be an exoplanetary astronomer,” says sity is their number. We’re talking exoplanets exoplanetary astronomer Jason Wright of Pennsyl- – planets around stars other than our Sun. And vania State University. “Things have really exploded they’re being discovered in Star Trek quantities: recently.” Proving the point is that a third of all 1692 as this article goes to press, and another 3845 abstracts at a recent meeting of the American Astro- unconfirmed candidates. nomical Society were related to exoplanets. The menagerie includes planets that are pink, This explosion is largely thanks to the Kepler space blue, brown or black. Some have been labelled hot observatory. -
2021 Review Guide Front Back
2021 IEEE Aerospace Conference AIAA - Technical Cosponsor March 6-13, 2021 Paper Review Guide 29th Annual Paper Review (Online) Oct. 1- Oct. 30, 2020 aeroconf.org 2021 IEEE Aerospace Conference Paper Review 2 2021 IEEE Aerospace Conference Paper Review 3 2021 IEEE Aerospace Conference Paper Review 4 All papers in this document are available for Online Review Track 2: Space Missions, Systems and Architectures; Peter Kahn; Steven Arnold Session 2.01 Deep Space, Earth and Discovery Missions; James Graf and Nick Chrissotimos 2.0101 Lucy Mission Ground System: Ground Readiness Testing during a Keri Siegel (General Dynamics C4S) Pandemic 2.0102 Redundancy in the Science Implementation of NASA’s Lucy Catherine Olkin (Southwest Research Mission to the Trojan Asteroids Institute) 2.0103 NASA's Lucy Mission to the Trojan Asteroids Harold Levison (Southwest Research Institute) 2.0105 Pointing Error Budget Development and Methodology on the Ashley Madni (Jet Propulsion Psyche Project Laboratory) 2.0106 NASA’s Lucy Mission: Encounter Simulations Julien Salmon (Southwest Research Institute) 2.0109 The Tandem Reconection and Cusp Electrodynamics Craig Kletzing (University of Iowa) Reconnaissance Satellites Mission 2.0116 Interstellar Mapping and Acceleration Probe (IMAP) Mission Douglas Eng (Johns Hopkins University Overview Applied Physics Laborator) 2.0119 IXPE Mission System and Development Status William Deininger (Ball Aerospace) Session 2.02 Future Space and Earth Science Missions; Patricia Beauchamp and Arthur Chmielewski 2.0201 NASA's Surface -
Two-Stage Attitude Control for Direct Imaging of Exoplanets with a Cubesat Telescope
Two-Stage Attitude Control for Direct Imaging of Exoplanets with a CubeSat Telescope Connor Beierlea, Andrew Nortonb, Bruce Macintoshb, and Simone D'Amicoa aSpace Rendezvous Laboratory, Stanford University, Stanford, CA bKavli Institute for Particle Astrophysics and Cosmology, Stanford University, Stanford, CA ABSTRACT This work outlines the design and development of a prototype CubeSat space telescope to directly image exo- planets and/or exozodiacal dust. This prototype represents the optical payload of the miniaturized distributed occulter/telescope (mDOT), a starshade technology demonstration mission combining a 2 meter scale microsatel- lite occulter and a 6U CubeSat telescope. Science requirements for the mDOT experiment are presented and translated into engineering requirements for the attitude determination and control subsystem (ADCS). The ADCS will utilize a triad of reaction wheels for coarse pointing and a tip tilt mirror for fine image stabilization down to the sub-arcsecond level. A two-stage attitude control architecture is presented to achieve precise point- ing necessary for stable acquisition of diffraction limited imagery. A multiplicative extended Kalman filter is utilized to estimate the inertial attitude of the vehicle and provide input into the aforementioned controller. A hardware-in-the-loop optical stimulator is used to stimulate the payload with scenes highly representative of the space environment from a radiometric and geometric stand point. Scenes rendered to the optical stimulator are synthesized in closed-loop based off a high-fidelity numerical simulation of the underlying disturbances, orbital and attitude dynamics. Performance of the two-stage attitude control loop is quantified and demonstrates the ability to achieve sub-arcsecond pointing using a telescope payload prototype. -
Exoplanet Exploration Program Updates
Exoplanet Exploration Program Updates Dr. Gary H. Blackwood, Program Manager Dr. Karl R. Stapelfeldt, Program Chief Scientist Jet Propulsion Laboratory California Institute of Technology July 29, 2018 ExoPAG 18, Cambridge MA © 2018 All rights reserved Artist concept of Kepler-16b Program Updates Program Investments LBTI Science Results and Implications Science Gap List NASA Exoplanet Exploration Program Astrophysics Division, NASA Science Mission Directorate NASA's search for habitable planets and life beyond our solar system Program purpose described in 2014 NASA Science Plan 1. Discover planets around other stars 2. Characterize their properties 3. Identify candidates that could harbor life ExEP serves the science community and NASA by implementing NASA’s space science vision for exoplanets https://exoplanets.nasa.gov WFIRST JWST2 PLATO Missions TESS Kepler LUVOIR5 CHEOPS 4 Spitzer Gaia Hubble1 Starshade HabEx5 CoRoT3 Rendezvous5 OST5 NASA Non-NASA Missions Missions W. M. Keck Observatory Large Binocular 1 NASA/ESA Partnership Telescope Interferometer NN-EXPLORE 2 NASA/ESA/CSA Partnership 3 CNES/ESA Ground Telescopes with NASA participation 5 4 ESA/Swiss Space Office 2020 Decadal Survey Studies NASA Exoplanet Exploration Program Space Missions and Mission Studies Communications K2 Probe-Scale Studies Starshade Coronagraph Supporting Research & Technology Key NASA Exoplanet Science Institute Sustaining Occulting Technology Development Research Masks Deformable Mirrors NN-EXPLORE Keck Single Archives, Tools, Sagan Fellowships, Aperture Professional Engagement Imaging & RV High-Contrast Imaging Deployable Starshades Large Binocular Telescope Interferometer https://exoplanets.nasa.gov 4 5 NASA Exoplanet Exploration Program Astrophysics Division, Science Mission Directorate Program Office (JPL) Changes since PM- Dr. G. Blackwood DPM- K. Short Chief Scientist – Dr.