Determination of Organophosphorus and Carbamate Insecticides in Food Samples by High-Performance Thin- Layer Chromatography Multi-Enzyme Inhibition Assay
Total Page:16
File Type:pdf, Size:1020Kb
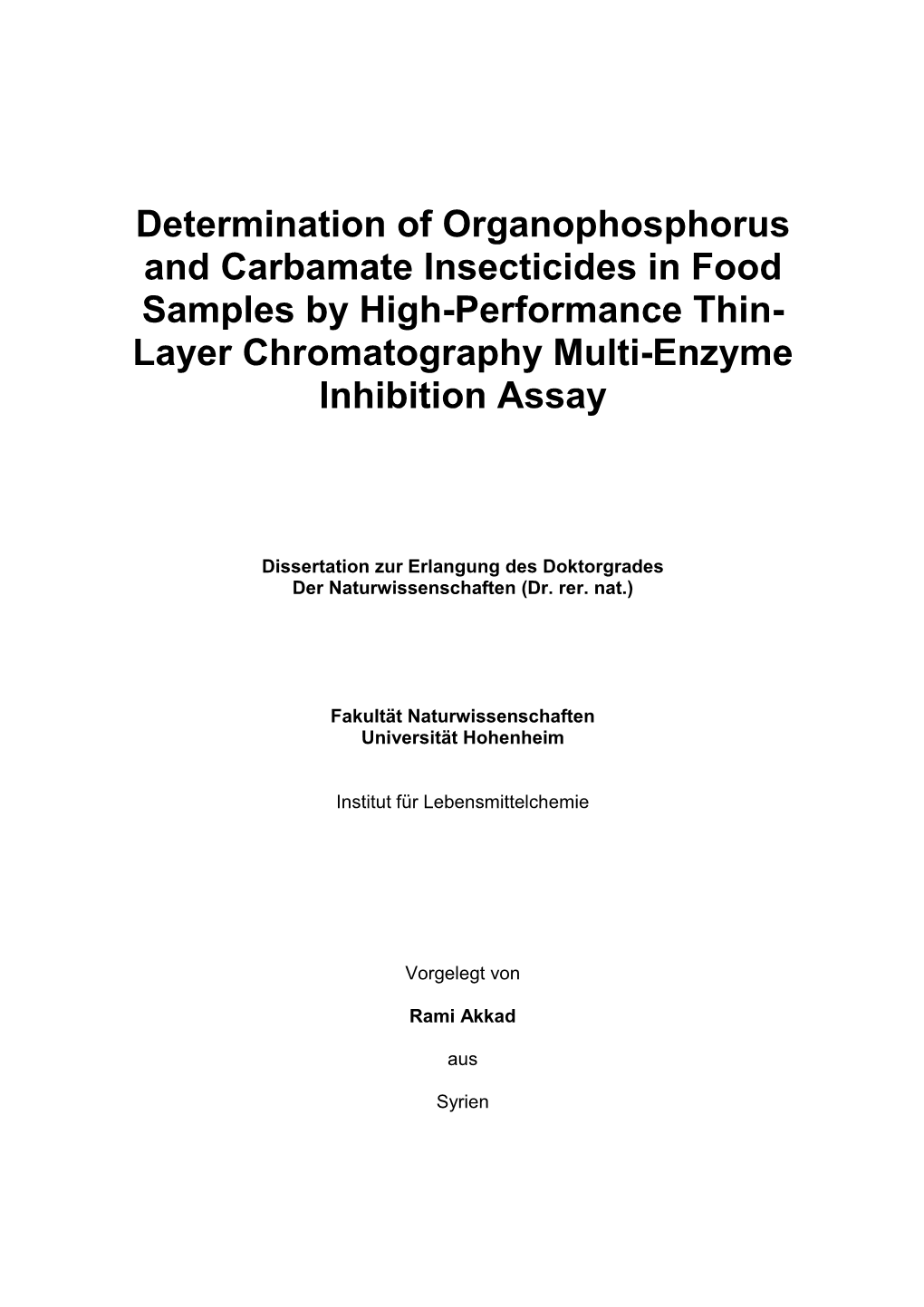
Load more
Recommended publications
-
Modifications on the Basic Skeletons of Vinblastine and Vincristine
Molecules 2012, 17, 5893-5914; doi:10.3390/molecules17055893 OPEN ACCESS molecules ISSN 1420-3049 www.mdpi.com/journal/molecules Review Modifications on the Basic Skeletons of Vinblastine and Vincristine Péter Keglevich, László Hazai, György Kalaus and Csaba Szántay * Department of Organic Chemistry and Technology, University of Technology and Economics, H-1111 Budapest, Szt. Gellért tér 4, Hungary * Author to whom correspondence should be addressed; E-Mail: [email protected]; Tel: +36-1-463-1195; Fax: +36-1-463-3297. Received: 30 March 2012; in revised form: 9 May 2012 / Accepted: 10 May 2012 / Published: 18 May 2012 Abstract: The synthetic investigation of biologically active natural compounds serves two main purposes: (i) the total synthesis of alkaloids and their analogues; (ii) modification of the structures for producing more selective, more effective, or less toxic derivatives. In the chemistry of dimeric Vinca alkaloids enormous efforts have been directed towards synthesizing new derivatives of the antitumor agents vinblastine and vincristine so as to obtain novel compounds with improved therapeutic properties. Keywords: antitumor therapy; vinblastine; vincristine; derivatives 1. Introduction Vinblastine (1) and vincristine (2) are dimeric alkaloids (Figure 1) isolated from the Madagaskar periwinkle plant (Catharantus roseus), exhibit significant cytotoxic activity and are used in the antitumor therapy as antineoplastic agents. In the course of cell proliferation they act as inhibitors during the metaphase of the cell cycle and by binding to the microtubules inhibit the development of the mitotic spindle. In tumor cells these agents inhibit the DNA repair and the RNA synthesis mechanisms, blocking the DNA-dependent RNA polymerase. Molecules 2012, 17 5894 Figure 1. -
Carbamate Pesticides Aldicarb Aldicarb Sulfoxide Aldicarb Sulfone
Connecticut General Statutes Sec 19a-29a requires the Commissioner of Public Health to annually publish a list setting forth all analytes and matrices for which certification for testing is required. Connecticut ELCP Drinking Water Analytes Revised 05/31/2018 Microbiology Total Coliforms Fecal Coliforms/ E. Coli Carbamate Pesticides Legionella Aldicarb Cryptosporidium Aldicarb Sulfoxide Giardia Aldicarb Sulfone Carbaryl Physicals Carbofuran Turbidity 3-Hydroxycarbofuran pH Methomyl Conductivity Oxamyl (Vydate) Minerals Chlorinated Herbicides Alkalinity, as CaCO3 2,4-D Bromide Dalapon Chloride Dicamba Chlorine, free residual Dinoseb Chlorine, total residual Endothall Fluoride Picloram Hardness, Calcium as Pentachlorophenol CaCO3 Hardness, Total as CaCO3 Silica Chlorinated Pesticides/PCB's Sulfate Aldrin Chlordane (Technical) Nutrients Dieldrin Endrin Ammonia Heptachlor Nitrate Heptachlor Epoxide Nitrite Lindane (gamma-BHC) o-Phosphate Metolachlor Total Phosphorus Methoxychlor PCB's (individual aroclors) Note 1 PCB's (as decachlorobiphenyl) Note 1 Demands Toxaphene TOC Nitrogen-Phosphorus Compounds Alachlor Metals Atrazine Aluminum Butachlor Antimony Diquat Arsenic Glyphosate Barium Metribuzin Beryllium Paraquat Boron Propachlor Cadmium Simazine Calcium Chromium Copper SVOC's Iron Benzo(a)pyrene Lead bis-(2-ethylhexyl)phthalate Magnesium bis-(ethylhexyl)adipate Manganese Hexachlorobenzene Mercury Hexachlorocyclopentadiene Molybdenum Nickel Potassium Miscellaneous Organics Selenium Dibromochloropropane (DBCP) Silver Ethylene Dibromide (EDB) -
COMBINED LIST of Particularly Hazardous Substances
COMBINED LIST of Particularly Hazardous Substances revised 2/4/2021 IARC list 1 are Carcinogenic to humans list compiled by Hector Acuna, UCSB IARC list Group 2A Probably carcinogenic to humans IARC list Group 2B Possibly carcinogenic to humans If any of the chemicals listed below are used in your research then complete a Standard Operating Procedure (SOP) for the product as described in the Chemical Hygiene Plan. Prop 65 known to cause cancer or reproductive toxicity Material(s) not on the list does not preclude one from completing an SOP. Other extremely toxic chemicals KNOWN Carcinogens from National Toxicology Program (NTP) or other high hazards will require the development of an SOP. Red= added in 2020 or status change Reasonably Anticipated NTP EPA Haz list COMBINED LIST of Particularly Hazardous Substances CAS Source from where the material is listed. 6,9-Methano-2,4,3-benzodioxathiepin, 6,7,8,9,10,10- hexachloro-1,5,5a,6,9,9a-hexahydro-, 3-oxide Acutely Toxic Methanimidamide, N,N-dimethyl-N'-[2-methyl-4-[[(methylamino)carbonyl]oxy]phenyl]- Acutely Toxic 1-(2-Chloroethyl)-3-(4-methylcyclohexyl)-1-nitrosourea (Methyl-CCNU) Prop 65 KNOWN Carcinogens NTP 1-(2-Chloroethyl)-3-cyclohexyl-1-nitrosourea (CCNU) IARC list Group 2A Reasonably Anticipated NTP 1-(2-Chloroethyl)-3-cyclohexyl-1-nitrosourea (CCNU) (Lomustine) Prop 65 1-(o-Chlorophenyl)thiourea Acutely Toxic 1,1,1,2-Tetrachloroethane IARC list Group 2B 1,1,2,2-Tetrachloroethane Prop 65 IARC list Group 2B 1,1-Dichloro-2,2-bis(p -chloropheny)ethylene (DDE) Prop 65 1,1-Dichloroethane -
Historical Perspectives on Apple Production: Fruit Tree Pest Management, Regulation and New Insecticidal Chemistries
Historical Perspectives on Apple Production: Fruit Tree Pest Management, Regulation and New Insecticidal Chemistries. Peter Jentsch Extension Associate Department of Entomology Cornell University's Hudson Valley Lab 3357 Rt. 9W; PO box 727 Highland, NY 12528 email: [email protected] Phone 845-691-7151 Mobile: 845-417-7465 http://www.nysaes.cornell.edu/ent/faculty/jentsch/ 2 Historical Perspectives on Fruit Production: Fruit Tree Pest Management, Regulation and New Chemistries. by Peter Jentsch I. Historical Use of Pesticides in Apple Production Overview of Apple Production and Pest Management Prior to 1940 Synthetic Pesticide Development and Use II. Influences Changing the Pest Management Profile in Apple Production Chemical Residues in Early Insect Management Historical Chemical Regulation Recent Regulation Developments Changing Pest Management Food Quality Protection Act of 1996 The Science Behind The Methodology Pesticide Revisions – Requirements For New Registrations III. Resistance of Insect Pests to Insecticides Resistance Pest Management Strategies IV. Reduced Risk Chemistries: New Modes of Action and the Insecticide Treadmill Fermentation Microbial Products Bt’s, Abamectins, Spinosads Juvenile Hormone Analogs Formamidines, Juvenile Hormone Analogs And Mimics Insect Growth Regulators Azadirachtin, Thiadiazine Neonicotinyls Major Reduced Risk Materials: Carboxamides, Carboxylic Acid Esters, Granulosis Viruses, Diphenyloxazolines, Insecticidal Soaps, Benzoyl Urea Growth Regulators, Tetronic Acids, Oxadiazenes , Particle Films, Phenoxypyrazoles, Pyridazinones, Spinosads, Tetrazines , Organotins, Quinolines. 3 I Historical Use of Pesticides in Apple Production Overview of Apple Production and Pest Management Prior to 1940 The apple has a rather ominous origin. Its inception is framed in the biblical text regarding the genesis of mankind. The backdrop appears to be the turbulent setting of what many scholars believe to be present day Iraq. -
WO 2018/005077 Al O O© O
(12) INTERNATIONAL APPLICATION PUBLISHED UNDER THE PATENT COOPERATION TREATY (PCT) (19) World Intellectual Property Organization International Bureau (10) International Publication Number (43) International Publication Date WO 2018/005077 Al 04 January 2018 (04.01.2018) W ! P O PCT (51) International Patent Classification: SC, SD, SE, SG, SK, SL, SM, ST, SV, SY, TH, TJ, TM, TN, A61K 31/78 (2006.01) C08J 7/04 (2006.01) TR, TT, TZ, UA, UG, US, UZ, VC, VN, ZA, ZM, ZW. C08G 59/77 (2006.01) (84) Designated States (unless otherwise indicated, for every (21) International Application Number: kind of regional protection available): ARIPO (BW, GH, PCT/US20 17/037 176 GM, KE, LR, LS, MW, MZ, NA, RW, SD, SL, ST, SZ, TZ, UG, ZM, ZW), Eurasian (AM, AZ, BY, KG, KZ, RU, TJ, (22) International Filing Date: TM), European (AL, AT, BE, BG, CH, CY, CZ, DE, DK, 13 June 2017 (13.06.2017) EE, ES, FI, FR, GB, GR, HR, HU, IE, IS, IT, LT, LU, LV, (25) Filing Language: English MC, MK, MT, NL, NO, PL, PT, RO, RS, SE, SI, SK, SM, TR), OAPI (BF, BJ, CF, CG, CI, CM, GA, GN, GQ, GW, (26) Publication Language: English KM, ML, MR, NE, SN, TD, TG). (30) Priority Data: 62/356,918 30 June 2016 (30.06.2016) US Published: — with international search report (Art. 21(3)) (71) Applicant: ELEMENTIS SPECIALTIES, INC. [US/US]; 469 Old Trenton Road, East Windsor, NJ 085 12 (US). (72) Inventors: IJDO, Wouter; 1224 Bridle Estates Dri ve, Yardley, PA 19067 (US). CHEN, Yanhui; 4 Hal- stead Place, Princeton, NJ 08540 (US). -
INDEX to PESTICIDE TYPES and FAMILIES and PART 180 TOLERANCE INFORMATION of PESTICIDE CHEMICALS in FOOD and FEED COMMODITIES
US Environmental Protection Agency Office of Pesticide Programs INDEX to PESTICIDE TYPES and FAMILIES and PART 180 TOLERANCE INFORMATION of PESTICIDE CHEMICALS in FOOD and FEED COMMODITIES Note: Pesticide tolerance information is updated in the Code of Federal Regulations on a weekly basis. EPA plans to update these indexes biannually. These indexes are current as of the date indicated in the pdf file. For the latest information on pesticide tolerances, please check the electronic Code of Federal Regulations (eCFR) at http://www.access.gpo.gov/nara/cfr/waisidx_07/40cfrv23_07.html 1 40 CFR Type Family Common name CAS Number PC code 180.163 Acaricide bridged diphenyl Dicofol (1,1-Bis(chlorophenyl)-2,2,2-trichloroethanol) 115-32-2 10501 180.198 Acaricide phosphonate Trichlorfon 52-68-6 57901 180.259 Acaricide sulfite ester Propargite 2312-35-8 97601 180.446 Acaricide tetrazine Clofentezine 74115-24-5 125501 180.448 Acaricide thiazolidine Hexythiazox 78587-05-0 128849 180.517 Acaricide phenylpyrazole Fipronil 120068-37-3 129121 180.566 Acaricide pyrazole Fenpyroximate 134098-61-6 129131 180.572 Acaricide carbazate Bifenazate 149877-41-8 586 180.593 Acaricide unclassified Etoxazole 153233-91-1 107091 180.599 Acaricide unclassified Acequinocyl 57960-19-7 6329 180.341 Acaricide, fungicide dinitrophenol Dinocap (2, 4-Dinitro-6-octylphenyl crotonate and 2,6-dinitro-4- 39300-45-3 36001 octylphenyl crotonate} 180.111 Acaricide, insecticide organophosphorus Malathion 121-75-5 57701 180.182 Acaricide, insecticide cyclodiene Endosulfan 115-29-7 79401 -
Index to the NLM Classification 2011
National Library of Medicine Classification 2011 Index Disease see Tyrosinemias 1-8 5,12-diHETE see Leukotriene B4 1,2-Benzopyrones see Coumarins 5,12-HETE see Leukotriene B4 1,2-Dibromoethane see Ethylene Dibromide 5-HT see Serotonin 1,8-Dihydroxy-9-anthrone see Anthralin 5-HT Antagonists see Serotonin Antagonists 1-Oxacephalosporin see Moxalactam 5-Hydroxytryptamine see Serotonin 1-Propanol 5-Hydroxytryptamine Antagonists see Serotonin Organic chemistry QD 305.A4 Antagonists Pharmacology QV 82 6-Mercaptopurine QV 269 1-Sar-8-Ala Angiotensin II see Saralasin 7S RNA see RNA, Small Nuclear 1-Sarcosine-8-Alanine Angiotensin II see Saralasin 8-Hydroxyquinoline see Oxyquinoline 13-cis-Retinoic Acid see Isotretinoin 8-Methoxypsoralen see Methoxsalen 15th Century History see History, 15th Century 8-Quinolinol see Oxyquinoline 16th Century History see History, 16th Century 17 beta-Estradiol see Estradiol 17-Ketosteroids WK 755 A 17-Oxosteroids see 17-Ketosteroids A Fibers see Nerve Fibers, Myelinated 17th Century History see History, 17th Century Aardvarks see Xenarthra 18th Century History see History, 18th Century Abate see Temefos 19th Century History see History, 19th Century Abattoirs WA 707 2',3'-Cyclic-Nucleotide Phosphodiesterases QU 136 Abbreviations 2,4-D see 2,4-Dichlorophenoxyacetic Acid Chemistry QD 7 2,4-Dichlorophenoxyacetic Acid General P 365-365.5 Organic chemistry QD 341.A2 Library symbols (U.S.) Z 881 2',5'-Oligoadenylate Polymerase see Medical W 13 2',5'-Oligoadenylate Synthetase By specialties (Form number 13 in any NLM -
Lifetime Organophosphorous Insecticide Use Among Private Pesticide Applicators in the Agricultural Health Study
Journal of Exposure Science and Environmental Epidemiology (2012) 22, 584 -- 592 & 2012 Nature America, Inc. All rights reserved 1559-0631/12 www.nature.com/jes ORIGINAL ARTICLE Lifetime organophosphorous insecticide use among private pesticide applicators in the Agricultural Health Study Jane A. Hoppin1, Stuart Long2, David M. Umbach3, Jay H. Lubin4, Sarah E. Starks5, Fred Gerr5, Kent Thomas6, Cynthia J. Hines7, Scott Weichenthal8, Freya Kamel1, Stella Koutros9, Michael Alavanja9, Laura E. Beane Freeman9 and Dale P. Sandler1 Organophosphorous insecticides (OPs) are the most commonly used insecticides in US agriculture, but little information is available regarding specific OP use by individual farmers. We describe OP use for licensed private pesticide applicators from Iowa and North Carolina in the Agricultural Health Study (AHS) using lifetime pesticide use data from 701 randomly selected male participants collected at three time periods. Of 27 OPs studied, 20 were used by 41%. Overall, 95% had ever applied at least one OP. The median number of different OPs used was 4 (maximum ¼ 13). Malathion was the most commonly used OP (74%) followed by chlorpyrifos (54%). OP use declined over time. At the first interview (1993--1997), 68% of participants had applied OPs in the past year; by the last interview (2005--2007), only 42% had. Similarly, median annual application days of OPs declined from 13.5 to 6 days. Although OP use was common, the specific OPs used varied by state, time period, and individual. Much of the variability in OP use was associated with the choice of OP, rather than the frequency or duration of application. -
Laboratory Test Report
Aromatic Plant Research Center 230 N 1200 E STE 100 Lehi, UT 84043 www.aromaticplant.org Laboratory Test Report SAMPLE NAME : Cypress CLIENT NAME : ACHS CLIENT LOT # : 36186C Column: ZB5 (60 m length × 0.25 mm inner diameter × 0.25 μm film thickness) Instrument: Shimadzu GCMS-QP2010 Ultra Carrier gas: Helium 80 psi Temperature ramp: 2 degrees Celsius per minute up to 260-degree Celsius Split ratio: 30:1 Sample preparation: 5% w/v solution with Dichloromethane Interpretation on sample: This analysis of Cypress essential oil meets the standard chemical profile of Cupressus sempervirens essential oil. Analyzed by: Dr. Prabodh Satyal Reviewed by: Ambika Poudel Issued Date: 9/25/2020 Copyright © 2020 by Aromatic Plant Research Center (APRC). All rights reserved. The information contained in this document may not be used, published or redistributed, including online, without the prior written consent of APRC. 1 / 4 24,426,457 Chromatogram Injection Volume Lot# Client Name Sample Name Sample Type Analyzed Analyzed by Sample Information www.aromaticplant.org UT Lehi, 84043 100 STE 230N E 1200 Center Aromatic Plant Research 10.856 Copyright © 2020 by Aromatic Plant Research Center (APRC). All rights reserved. Copyright information The All (APRC). rights reserved. this document contained in Center may © 2020by Aromatic Plantnot be used, published Research or 11.74611.898 13.37013.18113.088 12.429 14.335 14.41714.716 15.343 16.015 16.405 16.628 17.032 17.18317.501 18.00417.910 17.812 20.0 18.866 19.30119.646 21.153 21.744 21.445 22.392 : : : 0.30 : : : : ACHS Cy Esse 9/25/2020 7:12:23 AM AM 7:12:23 9/25/2020 Dr. -
N-Methyl Carbamate Cumulative Risk Assessment: Pilot Cumulative Analysis
UNITED STATES ENVIRONMENTAL PROTECTION AGENCY WASHINGTON, D.C. 20460 OFFICE OF PREVENTION, PESTICIDES, AND TOXIC SUBSTANCES April 15, 2005 MEMORANDUM SUBJECT: Transmittal of Meeting Minutes of the FIFRA Scientific Advisory Panel Meeting Held February 15 - 18, 2005 on the N-methyl Carbamate Cumulative Risk Assessment: Pilot Cumulative Analysis TO: James J. Jones, Director Office of Pesticide Programs FROM: Myrta R. Christian, Designated Federal Official Joseph E. Bailey, Designated Federal Official FIFRA Scientific Advisory Panel Office of Science Coordination and Policy THRU: Larry C. Dorsey, Executive Secretary FIFRA Scientific Advisory Panel Office of Science Coordination and Policy Clifford J. Gabriel, Director Office of Science Coordination and Policy Attached, please find the meeting minutes of the FIFRA Scientific Advisory Panel open meeting held in Arlington, Virginia on February 15 - 18, 2005. This report addresses a set of scientific issues being considered by the Environmental Protection Agency pertaining to the N- methyl carbamate cumulative risk assessment: pilot cumulative analysis. Attachment 1 of 113 cc: Susan Hazen Margaret Schneider Anne Lindsay Margie Fehrenbach Janet Andersen Debbie Edwards Steven Bradbury William Diamond Arnold Layne Tina Levine Lois Rossi Frank Sanders Richard Keigwin Randolph Perfetti William Jordan Douglas Parsons Enesta Jones Vanessa Vu (SAB) Anna Lowit David J. Miller Nelson Thurman Dirk Young David Hrdy Jeff Evans Steve Nako Stephanie Padilla R. Woodrow Setzer Ginger Moser Miles Okino Jerry Blancato Fred Power Curtis Dary Tom Nolan, USGS OPP Docket 2 of 113 FIFRA Scientific Advisory Panel Members Stephen M. Roberts, Ph.D. (Chair of the FIFRA SAP) Janice E. Chambers, Ph.D. H. Christopher Frey, Ph.D. -
Liquid and Gas Chromatographic Multi-Residue Pesticide Determination in Animal Tissues
Pestic. Sci. 1997, 49,56È64 Liquid and Gas Chromatographic Multi-residue Pesticide Determination in Animal Tissues Aurora Navas Dj az,* Angeles Garcj a Pareja & Francisco Garcj aSanchez Departamento de Qu•mica Anal•tica, Facultad de Ciencias, Universidad de Malaga, 29071-Malaga, Spain (Received 13 June 1995; revised version received 17 April 1996; accepted 16 August 1996) Abstract: A liquid chromatography multi-residue method with photometric detection has been developed. The method is applicable to the quantitative deter- mination of organochlorine (tetradifon, dicofol, chlorfenson, chlorobenzilate), organophosphorus (fenitrothion, azinphos-ethyl) and carbamate (pirimicarb) pesticides in animal tissues. The extracted residues are cleaned up by gel- permeation chromatography. A further fractionation on silica Sep-Pack car- tridges is included in the procedure. A gas chromatographic method with electron-capture detection for the analysis of the same pesticides was carried out and the results in the two cases compared. Lower detection and quantitation limits and similar recoveries of pesticides from spiked pig liver and brain samples were obtained by the LC method. Key words: liquid chromatography, gas chromatography, multi-residue pesti- cides, animal tissues 1 INTRODUCTION by various element-sensitive detectors, leading to reli- ance on mass spectrometry because of its ability to help Pest control in modern agriculture includes treatment to deÐne the structure. This has especially been true in of crops pre- and post-harvest with a variety of chemi- the case of the combination of gas chromatography cals, such as herbicides and insecticides in the pre- with mass spectrometry (GC/MS). One of the obvious harvest stage and with fungicides and rodenticides in Ðelds of application for this technique was the analysis the storage stage of the total harvest process. -
4C Pesticide Lists
4C PESTICIDE LISTS Version 4.0 II 4C PESTICIDE LISTS Copyright notice © 2020 4C Services GmbH This document is protected by copyright. It is freely available from the 4C website or upon request. No part of this copyrighted document may be changed or amended. The document may not be duplicated or copied in any form or by any means for commercial purpose without permission of 4C Services. Document Title: 4C Pesticide Lists Version 4.0 Valid from: 01 July 2020 III Content List of Tables ........................................................................................................................ IV Abbreviations ....................................................................................................................... IV 4C PESTICIDE LISTS 1 Introduction ................................................................................................................... 5 2 Selection Criteria Used for the 4C Pesticide Lists .......................................................... 5 3 4C Red List Pesticides: 4C Code of Conduct Requirements and Actions to be Promoted .................................. 6 4 4C Yellow List Pesticides: 4C Code of Conduct Requirements and Actions to be Promoted .................................. 7 © 4C Services GmbH IV List of Tables Table 1: 4C list of unacceptable pesticides ............................................................................ 8 Table 2: 4C red pesticide list ................................................................................................. 9 Table