Internal Structure and Palsa Development at Orravatnsrústir
Total Page:16
File Type:pdf, Size:1020Kb
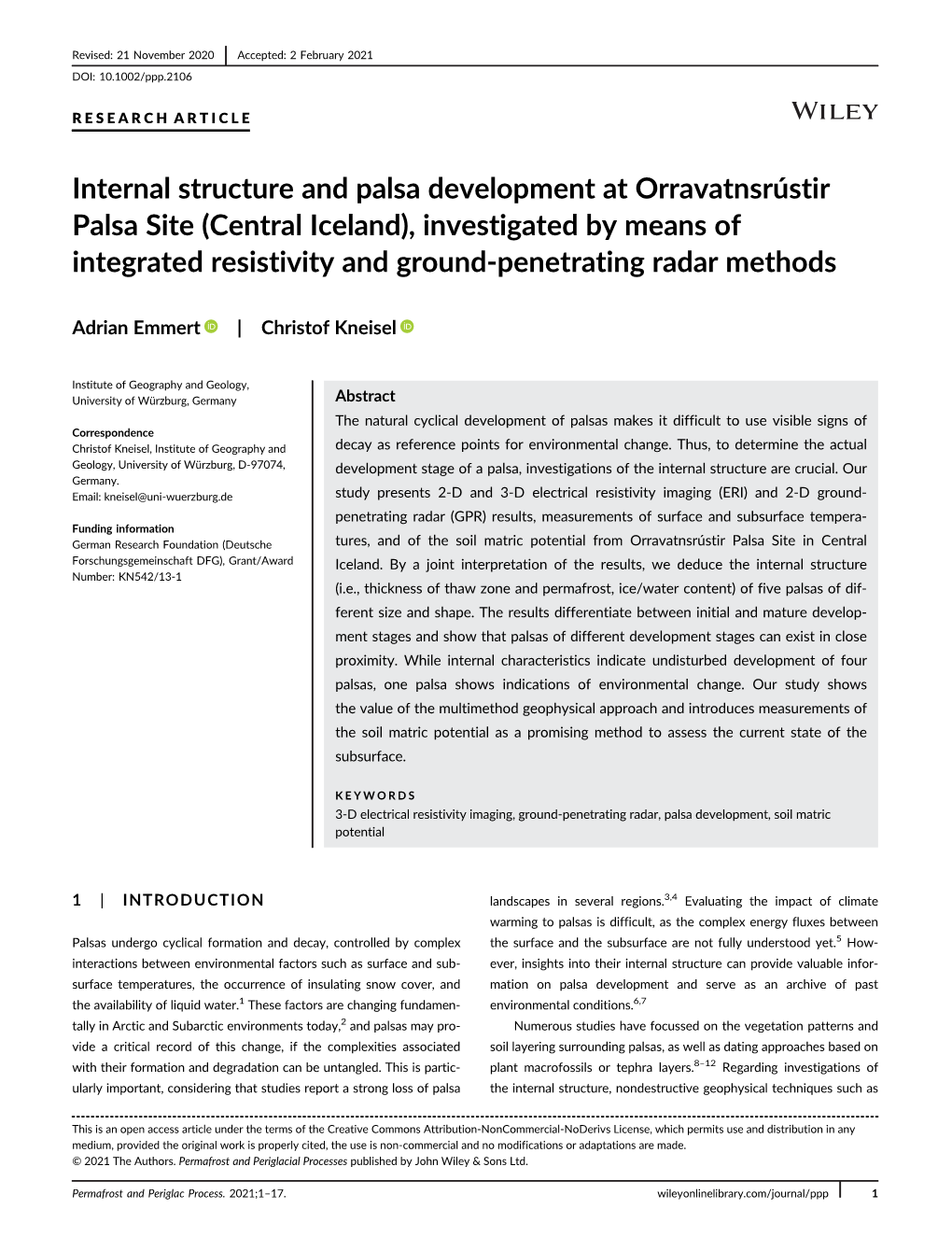
Load more
Recommended publications
-
High-Mountain Permafrost in the Austrian Alps (Europe)
HIGH-MOUNTAIN PERMAFROST IN THE AUSTRIAN ALPS (EUROPE) Gerhard Karl Lieb Institute of Geography University of Graz Heinrichstrasse 36 A-8010 Graz e-mail: [email protected] Abstract Permafrost research in the Austrian Alps (Eastern Alps) is based on a variety of methods, including at large scales, the measurement of the temperature of springs and of the base of winter snow cover, and at small scales, mainly an inventory of some 1450 rock glaciers. Taking all the information available into consideration, the lower limit of discontinuous permafrost is situated near 2500 m in most of the Austrian Alps. These results can be used for modelling the permafrost distribution within a geographical information system. Detailed investi- gations were carried out in the Doesen Valley (Hohe Tauern range) using additional methods, including several geophysical soundings. In this way, realistic estimates of certain permafrost characteristics and the volume of a large active rock glacier (some 15x106m3) were possible. This rock glacier has been chosen as a monitoring site to observe the effects of past and future climatic change. Introduction snow cover (BTS) and geophysical soundings, such as seismic, geoelectric, electromagnetic and ground pene- Although mountain permafrost in the Austrian Alps trating radar surveys have been published (survey and has caused construction problems and damage to buil- references in Lieb, 1996). The best results for mapping dings at several high-altitude locations, specific investi- the mere existence of permafrost were obtained by mea- gations of permafrost did not start until 1980. Since suring spring temperatures and BTS, both procedures then, studies of the distribution and certain characteris- being easily applicable and providing quite accurate tics of permafrost have been carried out at a number of interpretation. -
Late Wisconsin Climate Inferences from Rock Glaciers in South-Central
LateWisconsin climatic inlerences from rock glaciers in south-centraland west-central New Mexico andeast-central Arizona byJohn W. Blagbrough, P0 Box8063, Albuquerque, NewMexico 87198 Abstract Inactive rock glaciersof late Wisconsin age occur at seven sites in south-central and west-central New Mexico and in east-centralArizona. They are at the base of steep talus in the heads of canyons and ravines and have surfacefeatures indicating they are ice-cemented (permafrost) forms that moved by the flow of interstitial ice. The rock glaciersindicate zones of alpine permafrost with lower levels that rise from approximately 2,400m in the east region to 2,950 m in the west. Within the zones the mean annual temperaturewas below freezing, and the climatewas marked by much diurnal freezing and thawing resulting in the production of large volumes of talus in favorableterrain. The snow cover was thin and of short duration, which fa- vored ground freezing and cryofraction. The rock glaciers in the east region occur near the late Wisconsin 0'C air isotherm and implv that the mean annual temperature was depressedapproximately 7 to 8'C during a periglacial episodein the late Wisconsin.A dry continental climate with a seasonaldistribution of precipitation similar to that of the present probably prevailed, and timberline former timberlines. may have been depresseda minimum of 1,240m. The rise in elevation of the rock glaciersfrom east to west acrossthe region is attributed to greater snowfall in west-centralNew Mexico and east-centralArizona, which reducedthe inten- sity and depth of ground freezing near the late Wisconsin 0"C air isotherm. -
Inventory and Distribution of Rock Glaciers in Northeastern Yakutia
land Article Inventory and Distribution of Rock Glaciers in Northeastern Yakutia Vasylii Lytkin Melnikov Permafrost Institute, Siberian Branch of the Russian Academy of Sciences, Yakutsk 677010, Russia; [email protected] Received: 2 September 2020; Accepted: 8 October 2020; Published: 10 October 2020 Abstract: Rock glaciers are common forms of relief of the periglacial belt of many mountain structures in the world. They are potential sources of water in arid and semi-arid regions, and therefore their analysis is important in assessing water reserves. Mountain structures in the north-east of Yakutia have optimal conditions for the formation of rock glaciers, but they have not yet been studied in this regard. In this article, for the first time, we present a detailed list of rock glaciers in this region. Based on geoinformation mapping using remote sensing data and field studies within the Chersky, Verkhoyansk, Momsky and Suntar-Khayata ranges, 4503 rock glaciers with a total area of 224.6 km2 were discovered. They are located within absolute altitudes, from 503 to 2496 m. Their average minimum altitude was at 1456 m above sea level, and the maximum at 1527 m. Most of these formations are located on the sides of the trough valleys, and form extended sloping types of rock glaciers. An assessment of the exposure of the slopes where the rock glaciers are located showed that most of the rock glaciers are facing north and south. Keywords: rock glacier; permafrost; inventory; northeastern Yakutia; remote sensing 1. Introduction The geography of distribution of rock glaciers is quite extensive. They are found in many mountainous regions of Europe, North and South America and Asia, including some circumpolar regions [1–18]. -
Understanding Rock Glacier Landform Evolution and Recent Changes from Numerical Flow Modeling
The Cryosphere, 10, 2865–2886, 2016 www.the-cryosphere.net/10/2865/2016/ doi:10.5194/tc-10-2865-2016 © Author(s) 2016. CC Attribution 3.0 License. Rock glaciers on the run – understanding rock glacier landform evolution and recent changes from numerical flow modeling Johann Müller, Andreas Vieli, and Isabelle Gärtner-Roer Department of Geography, University of Zurich, Zurich, 8004, Switzerland Correspondence to: Johann Müller ([email protected]) Received: 4 February 2016 – Published in The Cryosphere Discuss.: 29 February 2016 Revised: 8 July 2016 – Accepted: 5 October 2016 – Published: 23 November 2016 Abstract. Rock glaciers are landforms that form as a result 1 Introduction of creeping mountain permafrost which have received con- siderable attention concerning their dynamical and thermal Rock glaciers and their dynamics have received much atten- changes. Observed changes in rock glacier motion on sea- tion in permafrost research and beyond, most prominently sonal to decadal timescales have been linked to ground tem- by the International Panel on Climate Change (IPCC) in the perature variations and related changes in landform geome- context of impacts of a warming climate on high mountain tries interpreted as signs of degradation due to climate warm- permafrost (IPCC, 2014). Time series of rock glacier move- ing. Despite the extensive kinematic and thermal monitoring ment in the European Alps indicate that acceleration in per- of these creeping permafrost landforms, our understanding mafrost creep in recent decades is related to an increase of the controlling factors remains limited and lacks robust in ground temperatures (Delaloye et al., 2010; PERMOS, quantitative models of rock glacier evolution in relation to 2013; Bodin et al., 2015). -
Patterned Ground on the Northern Side of the West Kunlun Mountains
Bu王1etinofGlacier Research7(1989)j45-j52 ム措 ⑥DataCenterforGlacierRcsearch,JapaneseSocietyofSnowandIce Patterned groundon the northern side ofthe West Kunlun Mountains,WeStern China ShujilWATAlandZHENGBenxing2 1DepartmentofGeography,FacultyofHumanitiesandSocialSciences,MieUniversity,Kamihama,Tsu,514Japan 2LarlZhouIn或ittlteOfGiacまologyandGeocryology,AcademiaSinica,Lanzbou,Ch重na (ReceivedI)ecember5,1988;RevisedmanuscriptreceivedJanuary23,1989) Abstract Mapping of earth hummocks,SOrted nets,SOrted stripes andlobes,Smallandlarge fissure mpolygons,andsomeotherperiglacialphenomenasuchasrockglacicrs,debris-mantledslopes,and involutions were condtlCted on the northern slopes of the West Kunlun Mountains,nOrthwestern margir10ftheTibctanPlateau,basedonfieldresearchin1987.Manydifferentkindsofpatterned groundoccurintbezoneabove5200minaititude,Whiiein汰ezonel)elow5280m,patternedgrotlnd occursonlyinlimitedがaces.Thisintensivedistributioninthehigherzonemaybeduetotheexistence ofcontinuouspermafrost,Whileinthelowerzonethepatternedgrounddevelopsinplaceswherethe soilmoistureconditionisfavorablc. l.lntroduction 1984;Cui,1985;Kuhle,1985;1987;Koizl】mi,1986; Wang,1987;Koazeetal.,1988),andthenorthernside Snowq andice十free areas of highmountain re- OftheHimalayas(軋爵Zhengetalリ1982).Incontrast, glOnSareSituatedinperiglacialenvirorlmentS,Where inthenorthwesternpart oftheTibetanPlateauin- 許Otlndたostdevelopsvar;ousperiglaciaipbeれOme11a CludingthelⅣestKunltlnMountains,inwhicbareathe Or主arld berleath the ground surねcet To assess払e periglacialdomain prestlmably extends very -
Aklisuktuk (Growing Fast) Pingo, Tuktoyaktuk Peninsula, Northwest Territories, Canada J
ARCTIC VOL, 34, NO. 3 (SEPTEMBER 1981). P. 270-273 Aklisuktuk (Growing Fast) Pingo, Tuktoyaktuk Peninsula, Northwest Territories, Canada J. ROSS MACKAY’ ABSTRACT. Field surveys have been carried out for the 1972 to 1979 period in order to study the growth of Aklisuktuk (Growing Fast) Pingo. The field surveys show that the top of the pingo was slowly subsiding dsring the seven-year survey period, possibly from a slow downslope glacier-like creep of the ice-rich overburden and ice core. The name “Aklisuktuk” probably dates back at least 200 years. The rapid growth which attracted attention was from accumulation of water in a large sub-pingo water lens. Key words: Aklisuktuk Pingo, sub-pingo water lens, pingo subsidence RÉSUMÉ: Des lev& sur le champs ont kt6 effectues entre1972 et 1979en vue d’ktudier le pingoAklisuktuk (“grandi vite”). Leslevks ont indique que le sommet du pingo s’est lentement affaisse au cours dela pkriodede lev6 de sept ans, possiblement B cause du lent avancement genre glacier du terrain de couverture riche en glace et du noyau de glace. Le nom “Aklisuktuk” date probablement d’il y a aumoins 200 ans. La croissance rapide qui a attire l’attention a kt6 causke par l’accumulation d’eau dans une grande poche au-dessous du pingo. Mots clés: Pingo Aklisuktuk, poche d’eau au-dessous du pingo, affaissement du pingo Traduit par Maurice Guibord, Le Centre Français, The University of Calgary. INTRODUCTION Pingos are ice-cored hills which can grow only in a permafrost environment. There are about 1450 pingos in the Tuktoyaktuk Peninsula area (Fig. -
The Dösen Rock Glacier in Central Austria: a Key Site for Multidisciplinary Long-Term Rock Glacier Monitoring in the Eastern Alps______
Austrian Journal of Earth Sciences Vienna 2017 Volume 110/2 xxx - xxx DOI: 10.17738/ajes.2017.0013 The Dösen Rock Glacier in Central Austria: A key site for multidisciplinary long-term rock glacier monitoring in the Eastern Alps_________________ Andreas KELLERER-PIRKLBAUER1)*), Gerhard Karl LIEB1), & Viktor KAUFMANN2) 1) Department of Geography and Regional Science, Working Group Alpine Landscape Dynamics (ALADYN), University of Graz, Heinrichstrasse 36, 8010 Graz, Austria; 2) Institute of Geodesy, Working Group Remote Sensing and Photogrammetry, Graz University of Technology, Steyrergasse 30, 8010 Graz, Austria; *) Corresponding author, [email protected] KEYWORDS rock glacier; monitoring; flow velocity; geophysics; ground temperature; Dösen Valley Abstract Rock glaciers are distinct landforms in high mountain environments indicating present or past permafrost conditions. Active rock glaciers contain permafrost and creep slowly downslope often forming typical flow structures with ridges and furrows related to compressional forces. Rock glaciers are widespread landforms in the Austrian Alps (c. 4600). Despite the high number of rock gla- ciers in Austria, only few of them have been studied in detail in the past. One of the best studied ones is the 950 m long Dösen Rock Glacier located in the Hohe Tauern Range. This rock glacier has been investigated since 1993 using a whole suite of field- based and remote sensing-based methods. Research focused on permafrost conditions and distribution, surface kinematics, inter- nal structure and possible age of the landform. Results indicate significant ground surface warming of the rock glacier body du- ring the period 2007-2015 accompanied by a general acceleration of the rock glacier surface flow velocity (max. -
Rock Glacier Dynamics Near the Lower Limit of Mountain Permafrost in the Swiss Alps
Rock Glacier Dynamics near the Lower Limit of Mountain Permafrost in the Swiss Alps Atsushi IKEDA A dissertation submitted to the Doctoral Program in Geoscience, the University of Tsukuba in partial fulfillment of the requirements for the degree of Doctor of Philosophy in Science January 2004 Contents Contents i Abstract iv List of figures vii List of tables x Chapter 1 Introduction 1 1.1. Mountain permafrost and rock glaciers 1 1.2. Internal structure and thermal conditions of rock glaciers 2 1.3. Dynamics of rock glaciers 4 1.4. Purpose of this study 6 Chapter 2 Study areas and sites 7 2.1. Upper Engadin and Davos 7 2.2. Büz rock glaciers 9 Chapter 3 Classification of rock glaciers 11 3.1. Classification by surface materials 11 3.2. Classification by activity 11 Chapter 4 Techniques 14 4.1. Mapping and measurements of terrain parameters 14 4.2. Temperature monitoring 15 4.2.1. Ground surface temperature 4.2.2. Subsurface temperature 4.3. Geophysical soundings 17 4.3.1. Hammer seismic sounding 4.3.2. DC resistivity imaging 4.4. Monitoring mass movements 19 i 4.4.1. Triangulation survey 4.4.2. Inclinometer measurement 4.5. Rockfall monitoring 20 Chapter 5 Contemporary dynamics of Büz rock glaciers 21 5.1. Internal structure 21 5.2. Thermal conditions 22 5.2.1. Snow thickness 5.2.2. Bottom temperature of the winter snow cover 5.2.3. Mean annual surface temperature 5.2.4. Subsurface temperature 5.3. Movement 27 5.3.1. Surface movement of BN rock glacier 5.3.2. -
Trends in Satellite Earth Observation for Permafrost Related Analyses—A Review
remote sensing Review Trends in Satellite Earth Observation for Permafrost Related Analyses—A Review Marius Philipp 1,2,* , Andreas Dietz 2, Sebastian Buchelt 3 and Claudia Kuenzer 1,2 1 Department of Remote Sensing, Institute of Geography and Geology, University of Wuerzburg, D-97074 Wuerzburg, Germany; [email protected] 2 German Remote Sensing Data Center (DFD), German Aerospace Center (DLR), Muenchner Strasse 20, D-82234 Wessling, Germany; [email protected] 3 Department of Physical Geography, Institute of Geography and Geology, University of Wuerzburg, D-97074 Wuerzburg, Germany; [email protected] * Correspondence: [email protected] Abstract: Climate change and associated Arctic amplification cause a degradation of permafrost which in turn has major implications for the environment. The potential turnover of frozen ground from a carbon sink to a carbon source, eroding coastlines, landslides, amplified surface deformation and endangerment of human infrastructure are some of the consequences connected with thawing permafrost. Satellite remote sensing is hereby a powerful tool to identify and monitor these features and processes on a spatially explicit, cheap, operational, long-term basis and up to circum-Arctic scale. By filtering after a selection of relevant keywords, a total of 325 articles from 30 international journals published during the last two decades were analyzed based on study location, spatio- temporal resolution of applied remote sensing data, platform, sensor combination and studied environmental focus for a comprehensive overview of past achievements, current efforts, together with future challenges and opportunities. The temporal development of publication frequency, utilized platforms/sensors and the addressed environmental topic is thereby highlighted. -
PERMAFROST Bob Carson June 2007
PERMAFROST Bob Carson June 2007 LAKE LINNEVATNET THE ACTIVE LAYER IS FROZEN ACTIVE LAYER PERMAFROST YUKON TERMS • PERMAFROST • PERIGLACIAL • \ • PATTERNED GROUND • POLYGONS • PALS • PINGO • ROCK GLACIER • THERMOKARST YAKIMA HILLS PROCESSES • FREEZE-THAW • FROST CRACK • FROST SHATTER • FROST HEAVE • FROST SHOVE • FROST SORT • CREEP • SOLIFLUCTION • NIVATION BEARTOOTH MOUNTAINS FROST CRACK • LOW-TEMPERATURE CONTRACTION ALASKA PHOTO BY RUTH SCHMIDT FROST SHATTER • WATER EXPANDS DURING FREEZING VATNAJOKULL KHARKHIRAA UUL FROST HEAVE FROST PUSH vs. FROST PULL CAIRNGORM FROST SHOVE GREENLAND PHOTO BY W.E. DAVIES FROST SORT SWEDISH LAPLAND PHOTO BY JAN BOELHOUWERS C R E E P SHARPE 1938 SOLIFLUCTION SOLIFLUCTION LOBES HANGAY NURUU NIVATION NIVATION HOLLOWS PALOUSE HILLS LANDFORMS WITH ICE ALASKA PHOTO BY SKIP WALKER AUFEIS KHARKHIRRA UUL HANGAY NURUU ICE WEDGES sis.agr.gc.ca/.../ground ICE-WEDGE POLYGONS res.agr.canada PALSEN HANGAY NURUU PALSEN FIELD OGILVIE MOUNTAINS PINGOES BEAUFORT COAST ALASKA PHOTO BY H.J.A. Berendsen ougseurope.org/rockon/surface/img PINGOES IN CANADIAN ARCTIC www.rekel.nl www.mbari.org www.arctic.uoguelph.ca VICTORIA ISLAND PHOTO BY A. L. WASHBURN ROCK GLACIERS GALENA CREEK ROCK GLACIERS GALENA CREEK ROCK GLACIERS GRAYWOLF RIDGE THERMOKARST YUKON THERMOKARST ALASKA ICE-WEDGE TRENCH YUKON ICE-WEDGE TRENCH ALASKA PHOTO BY JOE MOORE BEADED DRAINAGE ALASKA PHOTO BY RUTH SCHMIDT THAW LAKES PRUDOE BAY THAW LAKES ALASKA PHOTO BY ART REMPEL MORE PERIGLACIAL LANDFORMS SPITSBERGEN PHOTO BY BEN SCHUPACK WHITMAN ‘07 BLOCK FIELDS RINGING ROCKS BLOCK SLOPES BLOCK FIELD TALUS BLOCK SLOPE ELKHORN MOUNTAINS BLOCK STREAMS SAN FRANCISCO MOUNTAINS 11 June 2007 BLOCK STREAMS HANGAY NURUU CRYOPLANATION TERRACES HANGAY NURUU CRYOPLANATION TERRACES NIVATION TOR SOLIFLUCTION HANGAY NURUU PATTERNED GROUND: COMPONENTS FINES STONES HANGAY NURUU STONES: PEBBLES COBBLES BOULDERS FINES: CLAY, SILT, SAND PATTERNED GROUND: HANGAY COMPONENTS NURUU PATTERNED GROUND: CLASSIFICATION • SLOPE: HORIZONTAL± vs. -
Cartographic Display of Hazard and Risk Assessment Results for the Eastern Part of the Permafrost Zone of Russia
The aim is the comparative assessment of the territories of The research is conducted within the framework of the RGS-RFBR project “Current status and dynamics of municipalities of Siberia and the Far East in relation to their current and the natural hazards affecting the present day and potential transportation network of Siberia and Far East” potential exposure to such dangerous natural processes. The latter include thermokarst, thermal erosion and thermal abrasion, frost (mapping) and RFBR 18-05-60080 heaving, frost cracking, icing, kurums and rock glaciers. The expected Dangerous nival-glacial and cryogenic processes and their impact on infrastructure in the Arctic result is a contribution to the development strategy of the automobile (Field investigations in the Arctic) and railways of the regions, with the dynamics of the listed dangerous natural processes accounted for. Spread, Duration and Repeatability of cryogenic processes in the Russian Arctic (Decree of the President of the Russian Federation “On Land Territories of the Arctic Zone of the Russian Federation”, May, 2, 2014) (*) – municipalities partly located southern of the polar circle Frost Cracking Kurums Frost Heaving Icing Thermokarst Thermoerosion&Thermoabrasion Subject of the Repeat Repeata Repeat Repeat Repeat Repeat Federal district Municipality, 2014 Spread Duration Spread Duration Spread Duration Spread Duration Spread Duration Spread Duration federation ability bility ability ability ability ability Sibirskiy federalnyy okrug Krasnoyarsk Krai Taimyr Dolgan-Nenets -
PERIGLACIAL PROCESSES & LANDFORMS Periglacial
PERIGLACIAL PROCESSES & LANDFORMS Periglacial processes all non-glacial processes in cold climates average annual temperature between -15°C and 2°C fundamental controlling factors are intense frost action & ground surface free of snow cover for part of year Many periglacial features are related to permafrost – permanently frozen ground Permafrost table: upper surface of permafrost, overlain by 0-3 m thick active layer that freezes & thaws on seasonal basis Effects of frost action & mass movements enhanced by inability of water released by thawing active layer to infiltrate permafrost 454 lecture 11 Temperature fluctuations in permafrost to about 20-30 m depth; zero annual amplitude refers to level at which temperature is constant mean annual ground T - 10° 0° + 10° C permafrost table maximum minimum annual T annual T level of zero annual amplitude Permafrost base of permafrost 454 lecture 11 Slumping streambank underlain by permafrost, northern Brooks Range, Alaska 454 lecture 11 Taliks: unfrozen ground around permafrost surface of ground active layer permafrost table (upper surface of permafrost) permafrost talik 454 lecture 11 Permafrost tends to mimic surface topography large small river lake large lake pf permafrost talik Effect of surface water on the distribution of permafrost – taliks underlie water bodies 454 lecture 11 Stream cutbank underlain by permafrost 454 lecture 11 Where annual temperature averages < 0°C, ground freezing during the winter goes deeper than summer thawing – each year adds an increment & the permafrost grows