Mass Spectrometry Methods for the Analysis Of
Total Page:16
File Type:pdf, Size:1020Kb
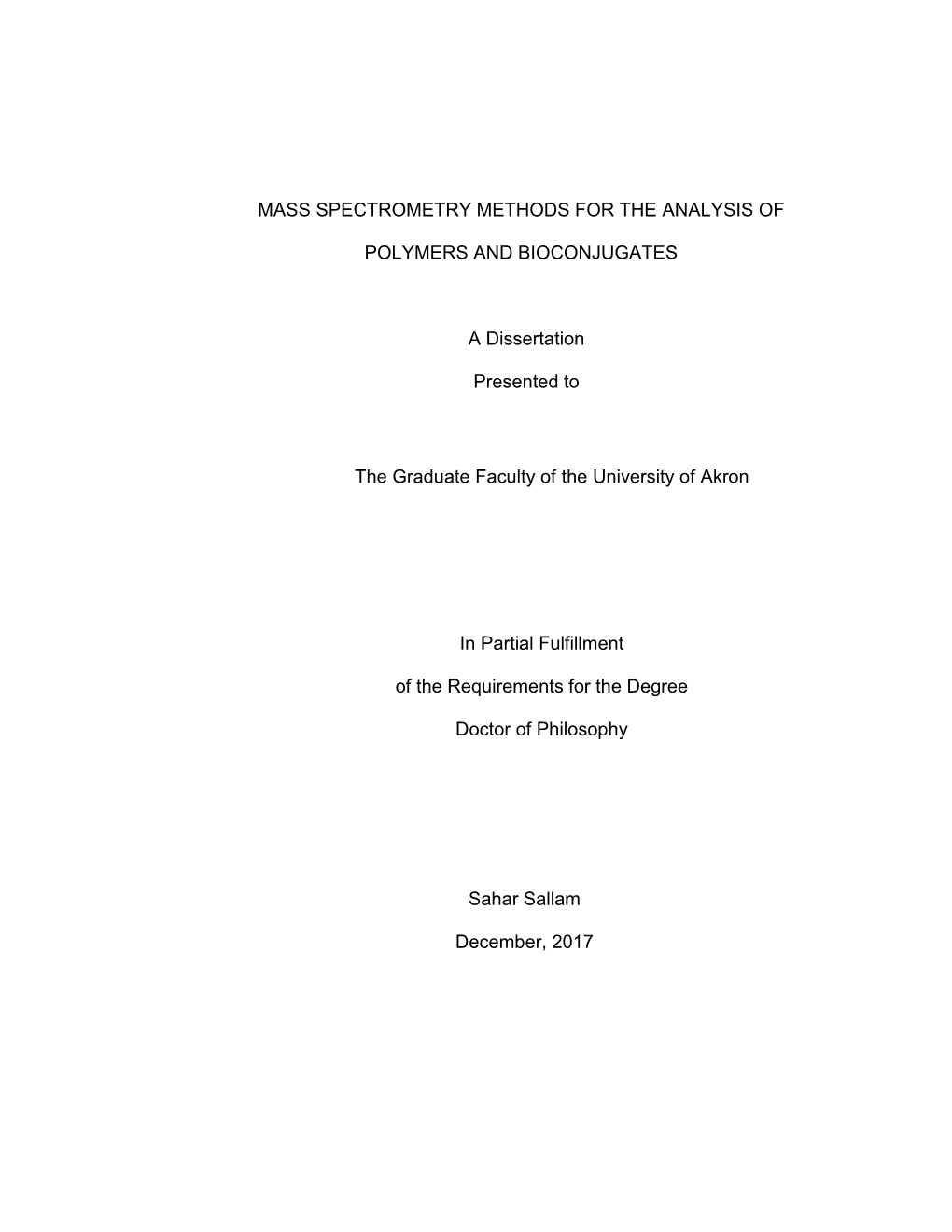
Load more
Recommended publications
-
Development of a Method for Xenon Determination in the Microstructure of High Burn-Up Nuclear Fuel
Diss. ETH No. 17527 Development of a Method for Xenon Determination in the Microstructure of High Burn-up Nuclear Fuel A dissertation submitted to the SWISS FEDERAL INSTITUTE OF TECHNOLOGY ZURICH for the degree of Dr. sc. ETH presented by MATTHIAS ISTVAN HORVATH Dipl. Phys. ETH born 24 August 1974 citizen of Châtillon (FR), Männedorf (ZH) - Switzerland citizen of Hungary accepted on the recommendation of Prof. Dr. D. Günther, examiner Prof. Dr. A. Wokaun, co-examiner Prof. Dr. Ch. Heinrich, co-examiner Dr. Ch. Hellwig, co-examiner 2008 II III Acknowledgements As many major work, this thesis could not have been performed and written, without the help and support of numerous people. This is especially true since this work was carried out at PSI and some experiments also at ETH combining different fields of research. I would like to send a special thank to my thesis advisor, Prof. Dr. Detlef Günther, and my supervisor Dr. Christian Hellwig for their support of my work at ETH and PSI. I am grateful to Dr. Marcel Guillong, with whom I got into the "world of LA-ICP-MS", and who’s experience was a great benefit. I would also to thank the members of the groups of Dr. Ines Günther-Leopold (Isotope and Element Analysis), Dr. Didier Gavillet (Surface and Solid State Materials), Daniel Kuster (Hot Cell Experiments), Dr. Johannes Bertsch (Core Safety Material Behavior) at PSI, and the trace element group of Prof. Dr. Detlef Günther at ETH. Without their support and the possibility using their infrastructure, this thesis could not be realized. -
Structural Amd Analytical Studies by Tandem Mass Spectrometry
STRUCTURAL AMD ANALYTICAL STUDIES BY TANDEM MASS SPECTROMETRY A Thesis submitted by TRACEY MADDEN for the degree of Doctor of Philosophy in the University of London Faculty of Science Department of Pharmaceutical Chemistry The School of Pharmacy University of London ProQuest Number: U556261 All rights reserved INFORMATION TO ALL USERS The quality of this reproduction is dependent upon the quality of the copy submitted. In the unlikely event that the author did not send a com plete manuscript and there are missing pages, these will be noted. Also, if material had to be removed, a note will indicate the deletion. uest ProQuest U556261 Published by ProQuest LLC(2017). Copyright of the Dissertation is held by the Author. All rights reserved. This work is protected against unauthorized copying under Title 17, United States C ode Microform Edition © ProQuest LLC. ProQuest LLC. 789 East Eisenhower Parkway P.O. Box 1346 Ann Arbor, Ml 48106- 1346 CONTENTS CHAPTER 1: INTRODUCTION 1.1 Tandem Mass Spectrometry ................... 1 REFERENCES ................................. 4 CHAPTER 2: THEORY 2.1 Mass Spectrometry ......................... 5 2.2 Formation of the Molecular Ion ............ 8 2.2.1 Vertical and Adiabatic Ionisation Potentials ......................... 9 2.2.2 Ionisation Efficiency Curve .......... 9 2.2.3 Appearance Energy ................... 10 2.3 Quasi Equilibrium Theory ................. 14 2.4 Fragmentation ............................ 16 2.4.1 Stevenson's Rule .................... 17 2.5 Rearrangement ............................ 19 2.6 Ion Stability ............................ 20 2.6.1 Stable Ions ......................... 20 2.6.2 Unstable Ions ....................... 21 2.6.3 Metastable Ions ..................... 21 2.6.3.1 Kinetic Energy Release ......... 22 2.6.3.2 Metastable Peak Shapes ....... -
Copyrighted Material
Contents Preface................................................................................................... xix Acknowledgments .............................................................................. xxiii Chapter 1 Introduction......................................................................... 1 I. Introduction ..........................................................................................................3 1. The Tools and Data of Mass Spectrometry...............................................4 2. The Concept of Mass Spectrometry..........................................................4 II. History ...................................................................................................................9 III. Some Important Terminology Used In Mass Spectrometry...........................22 1. Introduction..............................................................................................22 2. Ions..........................................................................................................22 3. Peaks ......................................................................................................23 4. Resolution and Resolving Power.............................................................25 IV. Applications........................................................................................................28 1. Example 1-1: Interpretation of Fragmentation Patterns (Mass Spectra) to Distinguish Positional Isomers .........................................................................29 -
Lecture 15 Spring 2005
Geol. 655 Isotope Geochemistry Lecture 15 Spring 2005 ANALYTICAL METHODOLOGY THE MASS SPECTROMETER In most cases, isotopic abundances are measured by mass spectrometry. The exceptions are, as we have seen, short-lived radioactive isotopes, the abundances of which are determined by measuring their decay rate, and in fission track dating, where the abundance of 238U is measured, in effect, by in- ducing fission. (Another exception is spectroscopic measurement of isotope ratios in stars. Frequencies of electromagnetic emissions of the lightest elements are sufficiently dependent on nuclear mass that emissions from different isotopes can be resolved. We will discuss this when we consider stable iso- topes.) A mass spectrometer is simply a device that can separate atoms or molecules according to their mass. There are a number of different kinds of mass spectrometers operating on different principles. Undoubtedly the vast majority of mass spectrometers are used by chemists for qualitative or quantita- tive analysis of organic compounds. We will focus exclusively, however, on mass spectrometers used for isotope ratio determination. Most isotope ratio mass spectrometers are of a similar design, the magnetic-sector, or Nier mass spectrometer*, a schematic of which is shown in Figure 15.1. It consists of three essential parts: an ion source, a mass analyzer and a detector. There are, however, several variations on the design of the Nier mass spectrometer. Some of these modifications relate to the spe- cific task of the instrument; others are evolutionary improvements. We will first consider the Nier mass spectrometer, and then briefly consider a few other kinds of mass spectrometers. -
Modern Mass Spectrometry
Modern Mass Spectrometry MacMillan Group Meeting 2005 Sandra Lee Key References: E. Uggerud, S. Petrie, D. K. Bohme, F. Turecek, D. Schröder, H. Schwarz, D. Plattner, T. Wyttenbach, M. T. Bowers, P. B. Armentrout, S. A. Truger, T. Junker, G. Suizdak, Mark Brönstrup. Topics in Current Chemistry: Modern Mass Spectroscopy, pp. 1-302, 225. Springer-Verlag, Berlin, 2003. Current Topics in Organic Chemistry 2003, 15, 1503-1624 1 The Basics of Mass Spectroscopy ! Purpose Mass spectrometers use the difference in mass-to-charge ratio (m/z) of ionized atoms or molecules to separate them. Therefore, mass spectroscopy allows quantitation of atoms or molecules and provides structural information by the identification of distinctive fragmentation patterns. The general operation of a mass spectrometer is: "1. " create gas-phase ions "2. " separate the ions in space or time based on their mass-to-charge ratio "3. " measure the quantity of ions of each mass-to-charge ratio Ionization sources ! Instrumentation Chemical Ionisation (CI) Atmospheric Pressure CI!(APCI) Electron Impact!(EI) Electrospray Ionization!(ESI) SORTING DETECTION IONIZATION OF IONS OF IONS Fast Atom Bombardment (FAB) Field Desorption/Field Ionisation (FD/FI) Matrix Assisted Laser Desorption gaseous mass ion Ionisation!(MALDI) ion source analyzer transducer Thermospray Ionisation (TI) Analyzers quadrupoles vacuum signal Time-of-Flight (TOF) pump processor magnetic sectors 10-5– 10-8 torr Fourier transform and quadrupole ion traps inlet Detectors mass electron multiplier spectrum Faraday cup Ionization Sources: Classical Methods ! Electron Impact Ionization A beam of electrons passes through a gas-phase sample and collides with neutral analyte molcules (M) to produce a positively charged ion or a fragment ion. -
C7895 Mass Spectrometry of Biomolecules Schedule of Lectures
C7895 Mass Spectrometry of Biomolecules Schedule of lectures For schedule, please see a separate file with the course outline. Jan Preisler Consulting The last lecture. Please contact me in advance to make an appointment. Chemistry Dept. 312A14, tel.: 54949 6629, [email protected] This material is just an outline; students are advised to print this outline and write down notes durin the lectures. The material will be updated during The course is focused on mass spectrometry of biomolecules, i.e. ionization the semester. techniques MALDI and ESI, modern mass analyzers, such as time-of-flight MS or ion traps and bioanalytical applications. However, the course covers much broader area, including inorganic ionization techniques, virtually all Supporting study material: types of mass analyzers and hardware in mass spectrometry. • J. Gross, Mass Spectrometry, 3rd ed. Springer-Verlag, 2017 • J. Greaves, J. Roboz: Mass Spectrometry for the Novice, CRC Press, 2013 • Edmond de Hoffmann, Vincent Stroobant: Mass Spectrometry: Principles and Applications, 3rd Edition, John Wiley & Sons, 2007 Mass spectrometry of biomolecules 2018 1 Mass spectrometry of biomolecules 2018 2 1 2 Content I. Introduction 1 II. Ionization methods and sample introduction III. Mass analyzers IV. Biological applications of MS V. Example problems Introduction to Mass spectrometry. Brief History of MS. A Survey of Methods and Instrumentation. Basic Concepts in MS: Resolution, Sensitivity. Isotope patterns of organic molecules. Ionization Techniques and Sample Introductin. Electron Impact Mass spectrometry of biomolecules 2018 3 Ionization (EI). Chemical Ionization (CI) 3 4 I. Introduction Study Material • Information sources about mass spectrometry Lecture notes • Brief history of mass spectrometry, a survey of methods and Advice: please take notes, but do not copy the slides; the English slides will instrumentation be provided at the end of the semester. -
Article Is Available Ca
Geochronology, 2, 343–354, 2020 https://doi.org/10.5194/gchron-2-343-2020 © Author(s) 2020. This work is distributed under the Creative Commons Attribution 4.0 License. Expanding the limits of laser-ablation U–Pb calcite geochronology Andrew R. C. Kylander-Clark Department of Earth Science, University of California, Santa Barbara, CA 93106, USA Correspondence: Andrew R. C. Kylander-Clark ([email protected]) Received: 30 May 2020 – Discussion started: 30 June 2020 Accepted: 20 September 2020 – Published: 23 November 2020 Abstract. U–Pb geochronology of calcite by laser-ablation 1 Introduction inductively coupled plasma mass spectrometry (LA-ICPMS) is an emerging field with potential to solve a vast array Calcite U–Pb geochronology by laser-ablation inductively of geologic problems. Because of low levels of U and Pb, coupled plasma mass spectrometry (LA-ICPMS) is a rela- measurement by more sensitive instruments, such as those tively new technique with untapped potential for solving nu- with multiple collectors (MCs), is advantageous. However, merous geochronologic problems from the timing of faulting whereas measurement of traditional geochronometers (e.g., (e.g., Roberts and Walker, 2016; Nuriel et al., 2017; Good- zircon) by MC-ICPMS has been limited by detection of the fellow et al., 2017) and the age of ore deposits (Burisch daughter isotope, U–Pb dating of calcite can be limited by et al., 2017) to paleoclimate, sedimentation, and diagene- detection of the parent isotope if measured on a Faraday sis (e.g., Mangenot et al., 2018; Rasbury et al., 1997; Hoff detector. The Nu P3D MC-ICPMS employs a new detec- et al., 1995; Winter and Johnson, 1995; Wang et al., 1998; tor array to measure all isotopes of interest on Daly detec- Rasbury et al., 1998). -
Phoenix with ATONA Brochure
The culmination of 40 years experience Thermal Ionization Mass Spectrometer in TIMS with ATONA Excellence in mass spectrometry Isotopx are the World class TIMS specialists. Phoenix TIMS Isotopx was formed in February 2008 as The Phoenix TIMS instrument is designed to heritage and a Management buyout (MBO) from GV measure isotope ratios of non-gaseous elements instruments. The MBO was in response with the highest sensitivity and precision. to Thermo Corporation’s purchase of GV knowledge instruments and the subsequent enquiry High sensitivity results from careful design of by the UK Competition Commission into the ion extraction optics combined with high the purchase. vacuum around the ionizing filament. High precision is also the result of highly We trace our heritage to the very first commercial TIMS, the MM30, launched by sensitive, low noise, stable detectors, that VG* Micromass in 1973. We are justifiably can measure extremely low ion currents from proud of this tradition, many of the nanoamps (nA, 1 x 10-9 A) to single ion subsequent instruments manufactured by detection (1.6 x 10-19 A). VG Isotopes, VG Isotech, Micromass and GV The Phoenix represents the ultimate instruments continue to be supported by Isotopx engineers even in some cases after combination of all these factors. more than 25 years of service. With the latest Phoenix the tradition of VG MM30 mass spectrometer, the worlds first multi-sample TIMS excellence is maintained. Operating at the highest levels of stability, sensitivity and precision, the Phoenix provides the current state of the art in isotope ratio analysis, Phoenix TIMS Design Features whatever the sample size. -
Expanded TITAN Plus
A 2-Way Daly-Type Beam Diagnostic System for TITAN EBIT By Cecilia Leung TRIUMF Undergraduate Summer Student Faculty of Science and Engineering Department of Physics and Astronomy York University August 2007 - 1 - CONTENTS Abstract . 3 1 Introduction 1.1 TRIUMF. 3 1.2 ISAC. 4 1.3 TITAN. 4 2 Beam Diagnostic System 2.1 Motivation. 8 2.2 Requirements . 8 2.2.1 Accommodate Beam from Two Ends. 9 2.2.2 Radiation Damage . 9 2.2.3 Perform high level Diagnostics . .10 2.2.4 Limitations. 10 2.3 The Daly Detector 2.3.1 The original Daly Detector . 11 2.4 The TITAN EBIT Daly-type design 2.4.1 Basic Components. 12 2.4.2 Principle of Operation . .13 2.4.3 Features . 15 3 Challenges and Considerations 3.1 Structural 3.1.1. Electric field shape . 16 3.1.2. Plate-grid separation. 17 3.1.3 Support structure . .18 3.2 Simulations of Environment with Expected beam parameters 3.2.1 Secondary e- distribution & trajectories . .18 3.2.2. MCP Efficiency in 5keV range. 19 3.2.3. Fringe field effects . 20 3.2.4. Ion Beam KE Energy Distribution . 20 3.2.5. EBIT Magnetic field influence . 21 4 Outlook 4.1 Alignment and Calibration. 22 4.2 Tests. 22 5 Conclusion . 23 Acknowledgments . 23 References . 25 Appendices: A GEM file for SIMION simulation. 27 B i. Prelliminary Design. .31 ii Ion Images Separation Interpolation to Find Optimal Plate Separation . 32 iii. Shield Shape . 35 - 2 - ABSTRACT A Daly-type diagnostic system was developed for the TRIUMF TITAN experiment to detect low-intensity ion beams based on imaging secondary electrons released upon radioactive ions striking an overhanging aluminum plate. -
© 2016 Lydia R. Cool All Rights Reserved
© 2016 LYDIA R. COOL ALL RIGHTS RESERVED IDENTIFYING AND DISTINGUISHING ISOMERS USING MASS SPECTROMETRY AND ION MOBILITY A Dissertation Presented to The Graduate Faculty of The University of Akron In Partial Fulfillment of the Requirements for the Degree Doctor of Philosophy Lydia R. Cool May, 2016 IDENTIFYING AND DISTINGUISHING ISOMERS USING MASS SPECTROMETRY AND ION MOBILITY Lydia R. Cool Dissertation Approved: Accepted: _____________________________ _____________________________ Advisor Department Chair Dr. Chrys Wesdemiotis Dr. Kim Calvo _____________________________ _____________________________ Committee Member Dean of the College Dr. Sailaja Paruchuri Dr. John Green _____________________________ _____________________________ Committee Member Dean of the Graduate School Dr. David Perry Dr. Chand Midha _____________________________ _____________________________ Committee Member Date Dr. Coleen Pugh _____________________________ Committee Member Dr. Claire Tessier ii ABSTRACT This dissertation focuses on the application of mass spectrometry (MS), tandem mass spectrometry (MS/MS), and ion mobility mass spectrometry (IM-MS) analysis of isomers. Chapter I gives an overview of the scope of the dissertation. Chapter II introduces mass spectrometry, including mass analyzers and ionization techniques. Chapter III discusses the instrumentation and materials used in this dissertation. Chapter IV discusses the analysis of five copolyesters. The first section of the chapter discusses two structural isomers synthesized using cyclohexane dicarboxylic acid (CHDA) and either 1,5-pentanediol (1,5-PED) or neopentyl glycol (NPG), viz. CHDA.NPG and CHDA.1,5-PED. Polyesters follow the 1,5-hydrogen rearrangement in MS/MS experiments, but CHDA.NPG cannot dissociate via this mechanism. A distinct, charge-induced fragmentation mechanism is proposed to operate in this case based on MS/MS fragmentation energetics and IM-MS results. -
Electron Transfer Dissociation and Collision-Induced Dissociation
ELECTRON TRANSFER DISSOCIATION AND COLLISION-INDUCED DISSOCIATION MASS SPECTROMETRY OF METALLATED OLIGOSACCHARIDES by RANELLE MARIE SCHALLER-DUKE CAROLYN J. CASSADY, COMMITTEE CHAIR JOHN B. VINCENT SHANE C. STREET ŁUKASZ CIEŚLA SHANLIN PAN A DISSERTATION Submitted in partial fulfillment of the requirements for the degree of Doctor of Philosophy in the Department of Chemistry and Biochemistry in the Graduate School of The University of Alabama TUSCALOOSA, ALABAMA 2019 Copyright Ranelle Marie Schaller-Duke 2019 ALL RIGHTS RESERVED ABSTRACT Investigations of metallated glycans through tandem mass spectrometry (MS/MS) can further the field of glycomics, the sequencing of the human glycome. The field is hindered by the lack of an analytical technique that can determine all the stereo-diverse features of carbohydrates. In this dissertation, electron transfer dissociation (ETD) and collision-induced dissociation (CID) are utilized with metal-adducted oligosaccharides to explore the potential of these techniques to sequence glycans. The resulting mass spectra provide significant insight and information about the structure of oligosaccharides and how to distinguish between these complicated isomeric species. Using univalent, divalent, and trivalent transition metal adducts is valuable to glycan analysis. The ETD process requires multiply charged ions, which do not form via protonation for neutral glycans, and CID of protonated glycans produces uninformative glycosidic bond cleavage. The univalent and trivalent metals investigated did not produce ions sufficient for ETD studies, but CID of the trivalent metal adducts showed significant fragmentation. Dissociation of [M + Met]2+ from the divalent metals formed various fragment ions with ETD producing more cross-ring and internal cleavages, which are necessary for structural analysis. -
Enhancement of Ion Transmission and Reduction of Background and Interferences in Inductively Coupled Plasma Mass Spectrometry Ke Hu Iowa State University
Iowa State University Capstones, Theses and Retrospective Theses and Dissertations Dissertations 1992 Enhancement of ion transmission and reduction of background and interferences in inductively coupled plasma mass spectrometry Ke Hu Iowa State University Follow this and additional works at: https://lib.dr.iastate.edu/rtd Part of the Analytical Chemistry Commons Recommended Citation Hu, Ke, "Enhancement of ion transmission and reduction of background and interferences in inductively coupled plasma mass spectrometry " (1992). Retrospective Theses and Dissertations. 10394. https://lib.dr.iastate.edu/rtd/10394 This Dissertation is brought to you for free and open access by the Iowa State University Capstones, Theses and Dissertations at Iowa State University Digital Repository. It has been accepted for inclusion in Retrospective Theses and Dissertations by an authorized administrator of Iowa State University Digital Repository. For more information, please contact [email protected]. INFORMATION TO USERS This manuscript has been reproduced from the microfilm master. UMI films the text directly from the original or copy submitted. Thus, some thesis and dissertation copies are in typewriter face, while others may be from any type of computer printer. The quality of this reproduction is dependent upon the quality of the copy submitted. Broken or indistinct print, colored or poor quality illustrations and photographs, print bleedthrough, substandard margins, and improper alignment can adversely affect reproduction. In the unlikely event that the author did not send UMI a complete manuscript and there are missing pages, these will be noted. Also, if unauthorized copyright material had to be removed, a note will indicate the deletion. Oversize materials (e.g., maps, drawings, charts) are reproduced by sectioning the original, beginning at the upper left-hand corner and continuing from left to right in equal sections with small overlaps.