1 Distribution and Seasonal Varibility in the Benthic Eukaryotic
Total Page:16
File Type:pdf, Size:1020Kb
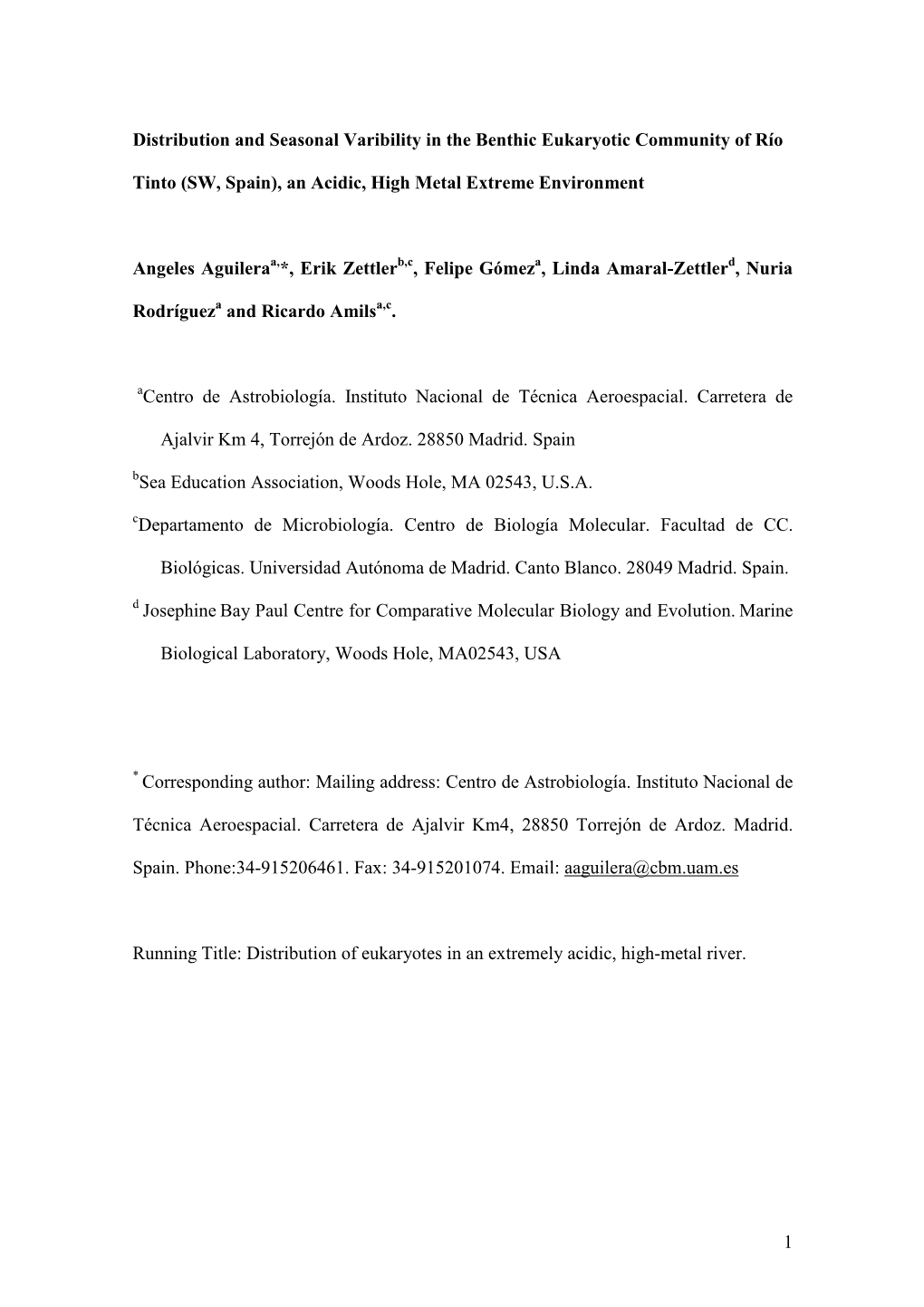
Load more
Recommended publications
-
Comparative Genomic View of the Inositol-1, 4, 5-Trisphosphate
Title Comparative Genomic View of The Inositol-1,4,5-Trisphosphate Receptor in Plants Author(s) Mikami, Koji Citation Journal of Plant Biochemistry & Physiology, 02(02) Issue Date 2014-9-15 Doc URL http://hdl.handle.net/2115/57862 Type article File Information comparative-genomic-view-of-the-inositoltrisphosphate-receptor.pdf Instructions for use Hokkaido University Collection of Scholarly and Academic Papers : HUSCAP Mikami, J Plant Biochem Physiol 2014, 2:3 Plant Biochemistry & Physiology http://dx.doi.org/10.4172/2329-9029.1000132 HypothesisResearch Article OpenOpen Access Access Comparative Genomic View of The Inositol-1,4,5-Trisphosphate Receptor in Plants Koji Mikami* Faculty of Fisheries Sciences, Hokkaido University, 3-1-1 Minato-cho, Hakodate 041 - 8611, Japan Abstract 2+ Terrestrial plants lack inositol-1,4,5-trisphosphate (IP3) receptor regulating transient Ca increase to activate 2+- cellular Ca dependent physiological events. To understand an evolutional route of the loss of the IP3 receptor gene, conservation of the IP3 receptor gene in algae was examined in silico based on the accumulating information of genomes and expression sequence tags. Results clearly demonstrated that the lack of the gene was observed in Rhodophyta, Chlorophyta except for Volvocales and Streptophyta. It was therefore hypothesized that the plant IP3 receptor gene was eliminated from the genome at multiple occasions; after divergence of Chlorophyta and Rhodophyta and of Chlorophyta and Charophyta. Keywords: Alga; Ca2+; Comparative genomics; Gene; Inositol-1,4,5- was probably lost from lineages of red algae and green algae except for trisphosphate receptor Volvocales (Figure 1). In fact, the genomes of unicellular Aureococcus anophagefferrens and multicellular Ectocarpus siliculosus carry an IP3 Abbreviations: DAG: Diacylglycerol; IP3: Inositol-1,4,5- receptor gene homologue (Figure 1). -
Dynamics of Phytoplankton Communities in Eutrophying Tropical Shrimp Ponds Affected by Vibriosis
1 Marine Pollution Bulletin Achimer September 2016, Volume 110, Issue 1, Pages 449-459 http://dx.doi.org/10.1016/j.marpolbul.2016.06.015 http://archimer.ifremer.fr http://archimer.ifremer.fr/doc/00343/45411/ © 2016 Elsevier Ltd. All rights reserved. Dynamics of phytoplankton communities in eutrophying tropical shrimp ponds affected by vibriosis Lemonnier Hugues 1, *, Lantoine François 2, Courties Claude 2, Guillebault Delphine 2, 3, Nézan Elisabeth 4, Chomérat Nicolas 4, Escoubeyrou Karine 5, Galinié Christian 6, Blockmans Bernard 6, Laugier Thierry 1 1 IFREMER LEAD, BP 2059, 98846 Nouméa cedex, New Caledonia, France 2 Sorbonne Universités, UPMC Univ Paris 06, UMR 8222, LECOB, Observatoire Océanologique, F- 66650 Banyuls/mer, France 3 Microbia Environnement, Observatoire Océanologique de Banyuls, 66650 Banyuls-sur mer, France 4 IFREMER, LER BO, Station de Biologie Marine, Place de la Croix, BP 40537, 29185 Concarneau Cedex, France 5 Sorbonne Universités, UPMC Univ Paris 06, CNRS, Plate-forme Bio2Mar, Observatoire Océanologique, F-66650 Banyuls/Mer, France 6 GFA, Groupement des Fermes Aquacoles, ORPHELINAT, 1 rue Dame Lechanteur, 98800 Nouméa cedex, New Caledonia, France * Corresponding author : Hugues Lemonnier, email address : [email protected] Abstract : Tropical shrimp aquaculture systems in New Caledonia regularly face major crises resulting from outbreaks of Vibrio infections. Ponds are highly dynamic and challenging environments and display a wide range of trophic conditions. In farms affected by vibriosis, phytoplankton biomass and composition are highly variable. These conditions may promote the development of harmful algae increasing shrimp susceptibility to bacterial infections. Phytoplankton compartment before and during mortality outbreaks was monitored at a shrimp farm that has been regularly and highly impacted by these diseases. -
Comparative Genomic View of the Inositol-1,4,5-Trisphosphate Receptor in Plants
Title Comparative Genomic View of The Inositol-1,4,5-Trisphosphate Receptor in Plants Author(s) Mikami, Koji Journal of Plant Biochemistry & Physiology, 02(02) Citation https://doi.org/10.4172/2329-9029.1000132 Issue Date 2014-9-15 Doc URL http://hdl.handle.net/2115/57862 Type article File Information comparative-genomic-view-of-the-inositoltrisphosphate-receptor.pdf Instructions for use Hokkaido University Collection of Scholarly and Academic Papers : HUSCAP Mikami, J Plant Biochem Physiol 2014, 2:3 Plant Biochemistry & Physiology http://dx.doi.org/10.4172/2329-9029.1000132 HypothesisResearch Article OpenOpen Access Access Comparative Genomic View of The Inositol-1,4,5-Trisphosphate Receptor in Plants Koji Mikami* Faculty of Fisheries Sciences, Hokkaido University, 3-1-1 Minato-cho, Hakodate 041 - 8611, Japan Abstract 2+ Terrestrial plants lack inositol-1,4,5-trisphosphate (IP3) receptor regulating transient Ca increase to activate 2+- cellular Ca dependent physiological events. To understand an evolutional route of the loss of the IP3 receptor gene, conservation of the IP3 receptor gene in algae was examined in silico based on the accumulating information of genomes and expression sequence tags. Results clearly demonstrated that the lack of the gene was observed in Rhodophyta, Chlorophyta except for Volvocales and Streptophyta. It was therefore hypothesized that the plant IP3 receptor gene was eliminated from the genome at multiple occasions; after divergence of Chlorophyta and Rhodophyta and of Chlorophyta and Charophyta. Keywords: Alga; Ca2+; Comparative genomics; Gene; Inositol-1,4,5- was probably lost from lineages of red algae and green algae except for trisphosphate receptor Volvocales (Figure 1). -
Rike Bacteria Protozoa Chromista Plantae
Svenska art projekt et Forskningsprioriteringar 2017 2017-03-21 RIKE UNDERRIKE INFRARIKE ÖVERSTAM DIVISION/STAM Ordning KLASS UNDERKLASS INFRAKLASS ÖVERORDNING Underordning "Infraordning" Familj SUBDIVISION/UNDERSTAM INFRASTAM ÖVERKLASS Överfamilj Underfamilj Forskningsprioritet Forskningsprioritet: 1= Mycket hög; 2= Hög; 3. Medelhög; 4= Låg; 5= Mycket låg BACTERIA 5 CYANOBACTERIA 5 PROTOZOA 5 EBRIOPHYCEAE 5 CILIOPHORA 5 FORAMINIFERA 5 CHOANOZOA 5 CHOANOFLAGELLIDA 5 MESOMYCETOZOEA 5 MYZOZOA 5 ELLOBIOPSEA 5 DINOPHYCEAE 5 EUGLENOZOA 5 EUGLENOPHYCEAE 5 Euglenales 5 Eutreptiales (syn. Sphenomonadales) 5 CERCOZOA 5 ASCETOSPOREA 5 PHYTOMYXEA (syn. PLASMODIOPHOROMYCETES) 5 LABYRINTHISTA ( syn. LABYRINTHULOMYCOTA) 5 APICOMPLEXA 5 PERCOLOZOA 5 HETEROLOBOSEA 5 Acrasida (syn. Acrasiomycetes) 5 SARCOMASTIGOPHORA 5 MYCETOZOA 3 DICTYOSTELIOMYCETES 5 MYXOMYCETES 3 PROTOSTELIOMYCETES 3 CHROMISTA CRYPTOPHYTA 5 HETEROKONTOPHYTA PHAEOPHYCEAE 3 Övriga klasser 5 BACILLARIOPHYTA 5 HAPTOPHYTA 5 HYPHOCHYTRIOMYCOTA 5 OOMYCOTA 3 PLANTAE BILIPHYTA RHODOPHYTA 2 VIRIDIPLANTAE CHLOROPHYTA CHLOROPHYTA CHLOROPHYCEAE Chaetophorales 3 Microsporales 3 1 Svenska art projekt et Forskningsprioriteringar 2017 2017-03-21 RIKE UNDERRIKE INFRARIKE ÖVERSTAM DIVISION/STAM Ordning KLASS UNDERKLASS INFRAKLASS ÖVERORDNING Underordning "Infraordning" Familj SUBDIVISION/UNDERSTAM INFRASTAM ÖVERKLASS Överfamilj Underfamilj Forskningsprioritet Oedogoniales 4 Övriga ordningar 5 ULVOPHYCEAE 3 TREBOUXIOPHYCEAE Prasiolales 3 Microthamniales 3 Övriga ordningar 5 PEDINOPHYCEAE 5 PRASINOPHYCEAE -
Kociolek University of Colorado Rev Standard Operating Procedures and Protocols for Algal Taxonomic Identification
Kociolek Rev University of Colorado Standard Operating Procedures and Protocols for 8 June, 2020 Algal Taxonomic Identification Table of Contents Section 1.0: Traceability of Analysis……………………………..…………………………………...2 A. Taxonomic Keys and References Used in the Identification of Soft-Bodied Algae and Diatoms.....2 B. Experts……………………………………………………………………………………………….6 C. Training Policy………………………………………………………………………………………7 Section 2.0: Procedures…………….……………………………………………………………………8 A. Sample Receiving……………………………………………………………………………………8 B. Storage……………………………………………………………………………………………….8 C. Processing……………………………………………………………………………………………8 i. Phytoplankton ii. Macroalgae iii. Periphyton iv. Preparation of Permanent Diatom Slides D. Analysis………………………………………………………………….…………………………14 i. Phytoplankton ii. Macroalgae iii. Periphyton iv.Identification and Enumeration Analysis of Diatoms E. Digital Image Reference Collection……………………………………………………………….....17 F. Development of List of Names……………………………………………………………………... 17 G. QA/QC Review……………………………………………………………………………………...17 H. Data Reporting……………………………………………………………………………………... 18 I. Archiving and Storage………………………………………………………………………………. 18 J. Shipment and Transport to Repository/BioArchive……………………………………………….... 18 K. Other Considerations……………………………………………………….………………………. 18 Section 3.0: QA/QC Protocols…………………………………………..………………………………19 Section 4.0: Relevant Literature………………………………………………………………………..20 1 Section 1.0 Traceability of Analysis A.Taxonomic Keys And References Used In The Identification Of Soft-Bodied Algae And Diatoms -
Compartmentalization of Mrnas in the Giant, Unicellular Green Algae
bioRxiv preprint doi: https://doi.org/10.1101/2020.09.18.303206; this version posted September 18, 2020. The copyright holder for this preprint (which was not certified by peer review) is the author/funder, who has granted bioRxiv a license to display the preprint in perpetuity. It is made available under aCC-BY-NC-ND 4.0 International license. 1 Compartmentalization of mRNAs in the giant, 2 unicellular green algae Acetabularia acetabulum 3 4 Authors 5 Ina J. Andresen1, Russell J. S. Orr2, Kamran Shalchian-Tabrizi3, Jon Bråte1* 6 7 Address 8 1: Section for Genetics and Evolutionary Biology, Department of Biosciences, University of 9 Oslo, Kristine Bonnevies Hus, Blindernveien 31, 0316 Oslo, Norway. 10 2: Natural History Museum, University of Oslo, Oslo, Norway 11 3: Centre for Epigenetics, Development and Evolution, Department of Biosciences, University 12 of Oslo, Kristine Bonnevies Hus, Blindernveien 31, 0316 Oslo, Norway. 13 14 *Corresponding author 15 Jon Bråte, [email protected] 16 17 Keywords 18 Acetabularia acetabulum, Dasycladales, UMI, STL, compartmentalization, single-cell, mRNA. 19 20 Abstract 21 Acetabularia acetabulum is a single-celled green alga previously used as a model species for 22 studying the role of the nucleus in cell development and morphogenesis. The highly elongated 23 cell, which stretches several centimeters, harbors a single nucleus located in the basal end. 24 Although A. acetabulum historically has been an important model in cell biology, almost 25 nothing is known about its gene content, or how gene products are distributed in the cell. To 26 study the composition and distribution of mRNAs in A. -
Peres Ck Dr Rcla.Pdf (2.769Mb)
UNIVERSIDADE ESTADUAL PAULISTA unesp “JÚLIO DE MESQUITA FILHO” INSTITUTO DE BIOCIÊNCIAS – RIO CLARO PROGRAMA DE PÓS-GRADUAÇÃO EM CIÊNCIAS BIOLÓGICAS (BIOLOGIA VEGETAL) TAXONOMIA, DISTRIBUIÇÃO AMBIENTAL E CONSIDERAÇÕES BIOGEOGRÁFICAS DE ALGAS VERDES MACROSCÓPICAS EM AMBIENTES LÓTICOS DE UNIDADES DE CONSERVAÇÃO DO SUL DO BRASIL CLETO KAVESKI PERES Tese apresentada ao Instituto de Biociências do Câmpus de Rio Claro, Universidade Estadual Paulista, como parte dos requisitos para obtenção do título de Doutor em Ciências Biológicas (Biologia Vegetal). Rio Claro Junho - 2011 CLETO KAVESKI PERES TAXONOMIA, DISTRIBUIÇÃO AMBIENTAL E CONSIDERAÇÕES BIOGEOGRÁFICAS DE ALGAS VERDES MACROSCÓPICAS EM AMBIENTES LÓTICOS DE UNIDADES DE CONSERVAÇÃO DO SUL DO BRASIL ORIENTADOR: Dr. CIRO CESAR ZANINI BRANCO Comissão Examinadora: Prof. Dr. Ciro Cesar Zanini Branco Departamento de Ciências Biológicas – Unesp/ Assis Prof. Dr. Carlos Eduardo de Mattos Bicudo Seção de Ecologia – Instituto de Botânica de São Paulo Prof. Dr. Orlando Necchi Júnior Departamento de Zoologia e Botânica/ IBILCE – UNESP/ São José do Rio Preto Profa. Dra. Ina de Souza Nogueira Departamento de Biologia – Universidade Federal de Goiás Profa. Dra. Célia Leite Sant´Anna Seção de Ficologia – Instituto de Botânica de São Paulo RIO CLARO 2011 AGRADECIMENTOS Muitas pessoas contribuíram com o desenvolvimento desse trabalho e com a minha formação pessoal e gostaria de deixar a todos meu reconhecimento e um sincero agradecimento. Porém, algumas pessoas/instituições foram fundamentais nestes quatro anos de doutorado, sendo imprescindível agradecê-las nominalmente: Aos meus pais, Euclinir e Lidia, por terem sempre acreditado em mim e por me incentivarem a continuar na área que escolhi. Agradeço imensamente pela melhor herança que uma pessoa pode receber que é o exemplo de humildade e dignidade que vocês têm. -
Freshwater Algae in Britain and Ireland - Bibliography
Freshwater algae in Britain and Ireland - Bibliography Floras, monographs, articles with records and environmental information, together with papers dealing with taxonomic/nomenclatural changes since 2003 (previous update of ‘Coded List’) as well as those helpful for identification purposes. Theses are listed only where available online and include unpublished information. Useful websites are listed at the end of the bibliography. Further links to relevant information (catalogues, websites, photocatalogues) can be found on the site managed by the British Phycological Society (http://www.brphycsoc.org/links.lasso). Abbas A, Godward MBE (1964) Cytology in relation to taxonomy in Chaetophorales. Journal of the Linnean Society, Botany 58: 499–597. Abbott J, Emsley F, Hick T, Stubbins J, Turner WB, West W (1886) Contributions to a fauna and flora of West Yorkshire: algae (exclusive of Diatomaceae). Transactions of the Leeds Naturalists' Club and Scientific Association 1: 69–78, pl.1. Acton E (1909) Coccomyxa subellipsoidea, a new member of the Palmellaceae. Annals of Botany 23: 537–573. Acton E (1916a) On the structure and origin of Cladophora-balls. New Phytologist 15: 1–10. Acton E (1916b) On a new penetrating alga. New Phytologist 15: 97–102. Acton E (1916c) Studies on the nuclear division in desmids. 1. Hyalotheca dissiliens (Smith) Bréb. Annals of Botany 30: 379–382. Adams J (1908) A synopsis of Irish algae, freshwater and marine. Proceedings of the Royal Irish Academy 27B: 11–60. Ahmadjian V (1967) A guide to the algae occurring as lichen symbionts: isolation, culture, cultural physiology and identification. Phycologia 6: 127–166 Allanson BR (1973) The fine structure of the periphyton of Chara sp. -
The Catalytic Core of DEMETER Guides Active DNA Demethylation in Arabidopsis
The catalytic core of DEMETER guides active DNA demethylation in Arabidopsis Changqing Zhanga,b,1, Yu-Hung Hunga,b,1, Hyun Jung Rimc,d,e,1, Dapeng Zhangf, Jennifer M. Frostg,2, Hosub Shinc,d,e, Hosung Jangc,d,e, Fang Liua,b,h, Wenyan Xiaof, Lakshminarayan M. Iyeri, L. Aravindi, Xiang-Qian Zhanga,b,j,3, Robert L. Fischerg,3, Jin Hoe Huhc,d,e,3, and Tzung-Fu Hsieha,b,3 aDepartment of Plant and Microbial Biology, North Carolina State University, Raleigh, NC 27695; bPlants for Human Health Institute, North Carolina State University, North Carolina Research Campus, Kannapolis, NC 28081; cDepartment of Plant Science, Seoul National University, 08826 Seoul, Republic of Korea; dResearch Institute for Agriculture and Life Sciences, Seoul National University, 08826 Seoul, Republic of Korea; ePlant Genomics and Breeding Institute, Seoul National University, 08826 Seoul, Republic of Korea; fDepartment of Biology, St. Louis University, St. Louis, MO 63103; gDepartment of Plant and Microbial Biology, University of California, Berkeley, CA 94720; hState Key Laboratory for Conservation and Utilization of Subtropical Agro-Bioresources, College of Agriculture, Guangxi University, 530004 Nanning, China; iNational Center for Biotechnology Information, National Library of Medicine, National Institutes of Health, Bethesda, MD 20894; and jCollege of Forestry and Landscape Architecture, South China Agricultural University, 510642 Guangzhou, China Contributed by Robert L. Fischer, July 12, 2019 (sent for review April 29, 2019; reviewed by James J. Giovannoni and Julie A. Law) The Arabidopsis DEMETER (DME) DNA glycosylase demethylates DME encodes a bifunctional 5mC DNA glycosylase/lyase that the maternal genome in the central cell prior to fertilization and is is essential for reproduction (9, 15). -
Identification of Algae in Water Supplies
Identification of Algae in Water Supplies Table of Contents Section I Introduction to the Algae by George Izaguirre Section II Review of Methods for Collection, Quantification and Identification of Algae by Miriam Steinitz-Kannan Section III Bibliography Section IV Key for the identification of the most common freshwater algae in water supplies Section V Photographs and descriptions of the most common genera of algae found in water supplies. Appendix A Figures A-1–A-6 Algae — AWWA Manual 7, Chapter 10 Continue Credits Copyright © 2002 American Water Works Association, all rights reserved. No copying of this informa- tion in any form is allowed without expressed written consent of the American Water Works Association. Disclaimer While AWWA makes every effort to ensure the accuracy of its products, it cannot guarantee 100% accuracy. In no event will AWWA be liable for direct, indirect, special, incidental, or consequential damages arising out of the use of information presented on this CD. In particular AWWA will not be responsible for any costs, including, but not limited to, those incurred as a result of lost revenue. In no event shall AWWA's liability exceed the amount paid for the purchase of this CD Identification of Algae in Water Supplies Section I Back to Table of Contents George Izaguirre The algae are a large and very diverse group of organisms that rangefrom minute single-celled forms to the giant marine kelps. They occupy a wide variety of habitats, including fresh water (lakes, reservoirs, and rivers), oceans, estuaries, moist soils, coastal spray zones, hot springs, snow fields and stone or concrete surfaces. -
The Catalytic Core of DEMETER Guides Active DNA Demethylation in Arabidopsis
The catalytic core of DEMETER guides active DNA demethylation in Arabidopsis Changqing Zhanga,b,1, Yu-Hung Hunga,b,1, Hyun Jung Rimc,d,e,1, Dapeng Zhangf, Jennifer M. Frostg,2, Hosub Shinc,d,e, Hosung Jangc,d,e, Fang Liua,b,h, Wenyan Xiaof, Lakshminarayan M. Iyeri, L. Aravindi, Xiang-Qian Zhanga,b,j,3, Robert L. Fischerg,3, Jin Hoe Huhc,d,e,3, and Tzung-Fu Hsieha,b,3 aDepartment of Plant and Microbial Biology, North Carolina State University, Raleigh, NC 27695; bPlants for Human Health Institute, North Carolina State University, North Carolina Research Campus, Kannapolis, NC 28081; cDepartment of Plant Science, Seoul National University, 08826 Seoul, Republic of Korea; dResearch Institute for Agriculture and Life Sciences, Seoul National University, 08826 Seoul, Republic of Korea; ePlant Genomics and Breeding Institute, Seoul National University, 08826 Seoul, Republic of Korea; fDepartment of Biology, St. Louis University, St. Louis, MO 63103; gDepartment of Plant and Microbial Biology, University of California, Berkeley, CA 94720; hState Key Laboratory for Conservation and Utilization of Subtropical Agro-Bioresources, College of Agriculture, Guangxi University, 530004 Nanning, China; iNational Center for Biotechnology Information, National Library of Medicine, National Institutes of Health, Bethesda, MD 20894; and jCollege of Forestry and Landscape Architecture, South China Agricultural University, 510642 Guangzhou, China Contributed by Robert L. Fischer, July 12, 2019 (sent for review April 29, 2019; reviewed by James J. Giovannoni and Julie A. Law) The Arabidopsis DEMETER (DME) DNA glycosylase demethylates DME encodes a bifunctional 5mC DNA glycosylase/lyase that the maternal genome in the central cell prior to fertilization and is is essential for reproduction (9, 15). -
An All-Taxa Biodiversity Inventory of the Huron Mountain Club
AN ALL-TAXA BIODIVERSITY INVENTORY OF THE HURON MOUNTAIN CLUB Version: August 2016 Cite as: Woods, K.D. (Compiler). 2016. An all-taxa biodiversity inventory of the Huron Mountain Club. Version August 2016. Occasional papers of the Huron Mountain Wildlife Foundation, No. 5. [http://www.hmwf.org/species_list.php] Introduction and general compilation by: Kerry D. Woods Natural Sciences Bennington College Bennington VT 05201 Kingdom Fungi compiled by: Dana L. Richter School of Forest Resources and Environmental Science Michigan Technological University Houghton, MI 49931 DEDICATION This project is dedicated to Dr. William R. Manierre, who is responsible, directly and indirectly, for documenting a large proportion of the taxa listed here. Table of Contents INTRODUCTION 5 SOURCES 7 DOMAIN BACTERIA 11 KINGDOM MONERA 11 DOMAIN EUCARYA 13 KINGDOM EUGLENOZOA 13 KINGDOM RHODOPHYTA 13 KINGDOM DINOFLAGELLATA 14 KINGDOM XANTHOPHYTA 15 KINGDOM CHRYSOPHYTA 15 KINGDOM CHROMISTA 16 KINGDOM VIRIDAEPLANTAE 17 Phylum CHLOROPHYTA 18 Phylum BRYOPHYTA 20 Phylum MARCHANTIOPHYTA 27 Phylum ANTHOCEROTOPHYTA 29 Phylum LYCOPODIOPHYTA 30 Phylum EQUISETOPHYTA 31 Phylum POLYPODIOPHYTA 31 Phylum PINOPHYTA 32 Phylum MAGNOLIOPHYTA 32 Class Magnoliopsida 32 Class Liliopsida 44 KINGDOM FUNGI 50 Phylum DEUTEROMYCOTA 50 Phylum CHYTRIDIOMYCOTA 51 Phylum ZYGOMYCOTA 52 Phylum ASCOMYCOTA 52 Phylum BASIDIOMYCOTA 53 LICHENS 68 KINGDOM ANIMALIA 75 Phylum ANNELIDA 76 Phylum MOLLUSCA 77 Phylum ARTHROPODA 79 Class Insecta 80 Order Ephemeroptera 81 Order Odonata 83 Order Orthoptera 85 Order Coleoptera 88 Order Hymenoptera 96 Class Arachnida 110 Phylum CHORDATA 111 Class Actinopterygii 112 Class Amphibia 114 Class Reptilia 115 Class Aves 115 Class Mammalia 121 INTRODUCTION No complete species inventory exists for any area.