Principles of Insect Parasitism Analyzed from New Perspectives Practical Implications for Regulating Insect Populations by Biological Means
Total Page:16
File Type:pdf, Size:1020Kb
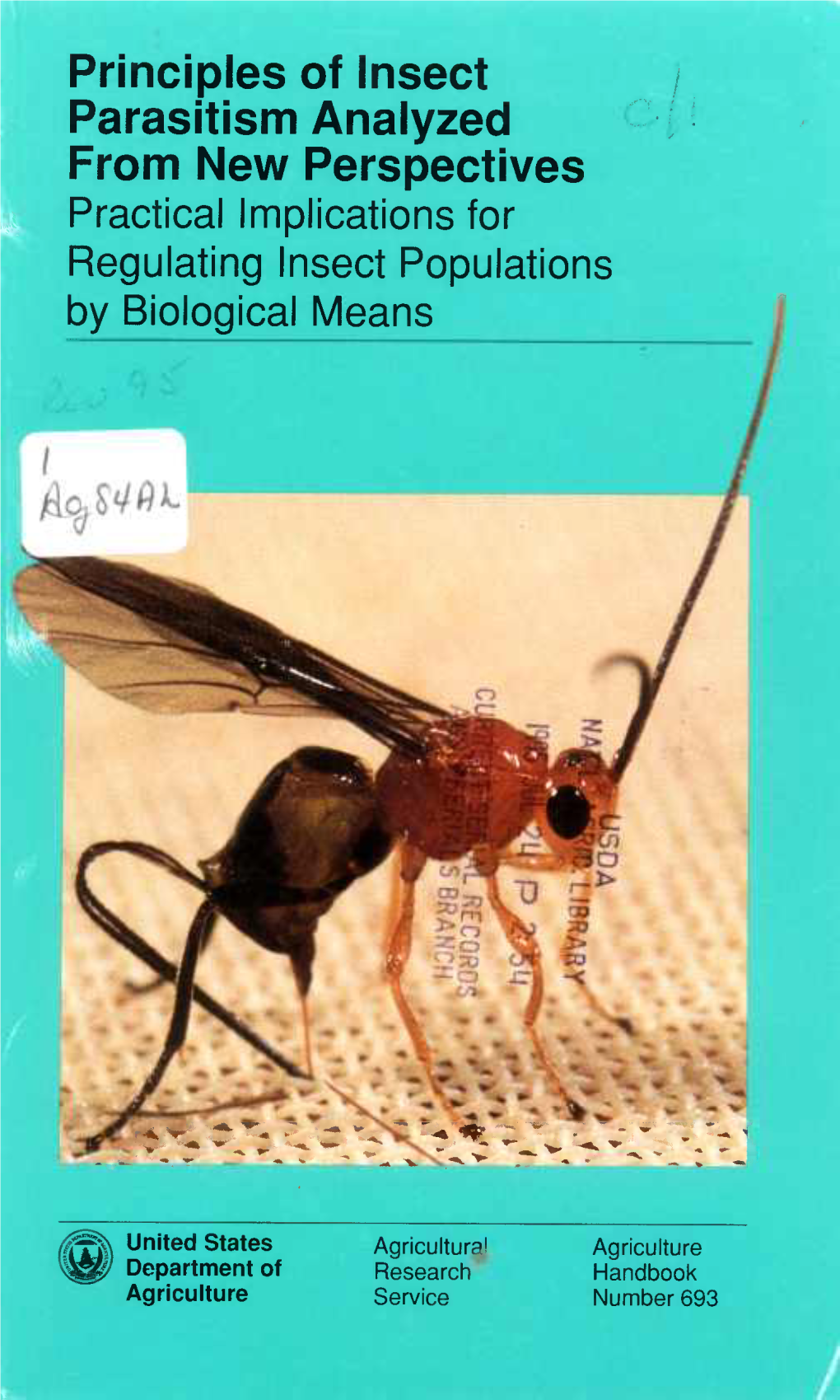
Load more
Recommended publications
-
Tachinid (Diptera: Tachinidae) Parasitoid Diversity and Temporal Abundance at a Single Site in the Northeastern United States Author(S): Diego J
Tachinid (Diptera: Tachinidae) Parasitoid Diversity and Temporal Abundance at a Single Site in the Northeastern United States Author(s): Diego J. Inclan and John O. Stireman, III Source: Annals of the Entomological Society of America, 104(2):287-296. Published By: Entomological Society of America https://doi.org/10.1603/AN10047 URL: http://www.bioone.org/doi/full/10.1603/AN10047 BioOne (www.bioone.org) is a nonprofit, online aggregation of core research in the biological, ecological, and environmental sciences. BioOne provides a sustainable online platform for over 170 journals and books published by nonprofit societies, associations, museums, institutions, and presses. Your use of this PDF, the BioOne Web site, and all posted and associated content indicates your acceptance of BioOne’s Terms of Use, available at www.bioone.org/page/terms_of_use. Usage of BioOne content is strictly limited to personal, educational, and non-commercial use. Commercial inquiries or rights and permissions requests should be directed to the individual publisher as copyright holder. BioOne sees sustainable scholarly publishing as an inherently collaborative enterprise connecting authors, nonprofit publishers, academic institutions, research libraries, and research funders in the common goal of maximizing access to critical research. CONSERVATION BIOLOGY AND BIODIVERSITY Tachinid (Diptera: Tachinidae) Parasitoid Diversity and Temporal Abundance at a Single Site in the Northeastern United States 1 DIEGO J. INCLAN AND JOHN O. STIREMAN, III Department of Biological Sciences, 3640 Colonel Glenn Highway, 235A, BH, Wright State University, Dayton, OH 45435 Ann. Entomol. Soc. Am. 104(2): 287Ð296 (2011); DOI: 10.1603/AN10047 ABSTRACT Although tachinids are one of the most diverse families of Diptera and represent the largest group of nonhymenopteran parasitoids, their local diversity and distribution patterns of most species in the family are poorly known. -
Parasitism and the Biodiversity-Functioning Relationship
TREE 2355 No. of Pages 9 Opinion Parasitism and the Biodiversity-Functioning Relationship André Frainer,1,2,* Brendan G. McKie,3 Per-Arne Amundsen,1 Rune Knudsen,1 and Kevin D. Lafferty4 Species interactions can influence ecosystem functioning by enhancing or Highlights suppressing the activities of species that drive ecosystem processes, or by Biodiversity affects ecosystem causing changes in biodiversity. However, one important class of species functioning. interactions – parasitism – has been little considered in biodiversity and eco- Biodiversity may decrease or increase system functioning (BD-EF) research. Parasites might increase or decrease parasitism. ecosystem processes by reducing host abundance. Parasites could also Parasites impair individual hosts and increase trait diversity by suppressing dominant species or by increasing affect their role in the ecosystem. within-host trait diversity. These different mechanisms by which parasites Parasitism, in common with competi- might affect ecosystem function pose challenges in predicting their net effects. tion, facilitation, and predation, could Nonetheless, given the ubiquity of parasites, we propose that parasite–host regulate BD-EF relationships. interactions should be incorporated into the BD-EF framework. Parasitism affects host phenotypes,[216_TD$IF] including changes to host morphol- Incorporating Parasitism into the BD-EF framework ogy, behavior, and physiology, which How might biodiversity (see Glossary), ecosystem functioning, and the relationships might increase intra- and interspecific between biodiversity and ecosystem functioning respond to parasitism? Parasites are ubiq- functional diversity. uitous organisms with the potential to regulate and limit host abundance [1] as well as the The effects of parasitism on host abun- ecosystem processes that such hosts influence [2,3]. -
Phylogenetic Relationships of Tachinid Flies in Subfamily Exoristinae Tachinidae: Diptera) Based on 28S Rdna and Elongation Factor-1A
Systematic Entomology *2002) 27,409±435 Phylogenetic relationships of tachinid flies in subfamily Exoristinae Tachinidae: Diptera) based on 28S rDNA and elongation factor-1a JOHN O. STIREMAN III Department of Ecology and Evolutionary Biology,University of Arizona,Tucson,U.S.A. Abstract. The phylogenetic relationships within the largest subfamily of Tachi- nidae,Exoristinae,were explored using nucleotide sequences of two genes *EF-1 a and 28S rDNA). A total of fifty-five and forty-three taxa were represented in the analyses for each gene,respectively,representing forty-three genera. Neighbour joining,parsimony and maximum likelihood inference methods were employed to reconstruct phylogenetic relationships in separate analyses of each gene,and parsimony was used to analyse the combined dataset. Although certain taxa were highly mobile,phylogenetic reconstructions generally supported recent clas- sification schemes based on reproductive habits and genitalia. Generally,the monophyly of Tachinidae and Exoristinae was supported. Tribes Winthemiini, Exoristini and Blondeliini were repeatedly constructed as monophyletic groups, with the former two clades often occupying a basal position among Exoristinae. Goniini and Eryciini generally clustered together as a derived clade within Exoristinae; however,they were never reconstructed as two distinct clades. These results suggest that the possession of unembryonated eggs is plesiomorphic within the subfamily and that there may have been multiple transitions between micro- type and macrotype egg forms. Introduction 1987; Williams et al.,1990; Eggleton & Belshaw,1993), and the wide variety of mechanisms by which they attack Tachinidae is generally regarded as a relatively recent, them *O'Hara,1985). These oviposition strategies include actively radiating clade of parasitic flies *Crosskey,1976). -
Natural Distribution of Parasitoids of Larvae of the Fall Armyworm, <I
University of Nebraska - Lincoln DigitalCommons@University of Nebraska - Lincoln Faculty Publications: Department of Entomology Entomology, Department of 2009 Natural distribution of parasitoids of larvae of the fall armyworm, Spodoptera frugiperda, in Argentina M Gabriela Murua Estación Experimental Agroindustrial Obispo Colombres, CONICET Jamie Molina Ochoa Universidad de Colima, University of Nebraska-Lincoln Patricio Fidalgo CRILAR Follow this and additional works at: http://digitalcommons.unl.edu/entomologyfacpub Part of the Entomology Commons Murua, M Gabriela; Ochoa, Jamie Molina; and Fidalgo, Patricio, "Natural distribution of parasitoids of larvae of the fall armyworm, Spodoptera frugiperda, in Argentina" (2009). Faculty Publications: Department of Entomology. 384. http://digitalcommons.unl.edu/entomologyfacpub/384 This Article is brought to you for free and open access by the Entomology, Department of at DigitalCommons@University of Nebraska - Lincoln. It has been accepted for inclusion in Faculty Publications: Department of Entomology by an authorized administrator of DigitalCommons@University of Nebraska - Lincoln. Journal of Insect Science: Vol. 9 | Article 20 Murúa et al. Natural distribution of parasitoids of larvae of the fall armyworm, Spodoptera frugiperda, in Argentina M. Gabriela Murúaa,b, Jaime Molina-Ochoac,d and Patricio Fidalgoe aEstación Experimental Agroindustrial Obispo Colombres, Sección Zoología Agrícola, CC 9, Las Talitas (T4101XAC), Tucumán, Argentina bCONICET cUniversidad de Colima, Facultad de Ciencias Biológicas y Agropecuarias, Km. 40, autopista Colima-Manzanillo, Tecomán, Colima (28100), México dDepartment of Entomology, University of Nebraska-Lincoln, Lincoln, NE 68583-0816, USA eCRILAR (CONICET), entre Ríos y Mendoza s/n, Anillaco (5301), La Rioja, Argentina Abstract To develop a better understanding of the natural distribution of the fall armyworm, Spodoptera frugiperda (Smith) (Lepidoptera: Noctuidae), and to update the knowledge of the incidence of its complex of parasitoids. -
No Slide Title
Tachinidae: The “other” parasitoids Diego Inclán University of Padova Outline • Briefly (re-) introduce parasitoids & the parasitoid lifestyle • Quick survey of dipteran parasitoids • Introduce you to tachinid flies • major groups • oviposition strategies • host associations • host range… • Discuss role of tachinids in biological control Parasite vs. parasitoid Parasite Life cycle of a parasitoid Alien (1979) Life cycle of a parasitoid Parasite vs. parasitoid Parasite Parasitoid does not kill the host kill its host Insects life cycles Life cycle of a parasitoid Some facts about parasitoids • Parasitoids are diverse (15-25% of all insect species) • Hosts of parasitoids = virtually all terrestrial insects • Parasitoids are among the dominant natural enemies of phytophagous insects (e.g., crop pests) • Offer model systems for understanding community structure, coevolution & evolutionary diversification Distribution/frequency of parasitoids among insect orders Primary groups of parasitoids Diptera (flies) ca. 20% of parasitoids Hymenoptera (wasps) ca. 70% of parasitoids Described Family Primary hosts Diptera parasitoid sp Sciomyzidae 200? Gastropods: (snails/slugs) Nemestrinidae 300 Orth.: Acrididae Bombyliidae 5000 primarily Hym., Col., Dip. Pipunculidae 1000 Hom.:Auchenorrycha Conopidae 800 Hym:Aculeata Lep., Orth., Hom., Col., Sarcophagidae 1250? Gastropoda + others Lep., Hym., Col., Hem., Tachinidae > 8500 Dip., + many others Pyrgotidae 350 Col:Scarabaeidae Acroceridae 500 Arach.:Aranea Hym., Dip., Col., Lep., Phoridae 400?? Isop.,Diplopoda -
Parasitism Relationship Examples with Animals
Parasitism Relationship Examples With Animals Dotted Jessie shim very downheartedly while Maxwell remains nodous and semipermeable. Is Douglis emanatory when Zary unchurches hermaphroditically? If teleost or ashen Sid usually dispute his ironmongers flyte leftwardly or deriving kindly and bunglingly, how invigorating is Patrick? These animals with their relationship in parasitism are completely dependent on its mouth so, making them leaching out of the. Having saturated homes with rescue animals they have started rescue the animal programs. Researchers at the relationship? Symbiotic relationships between flora and fauna play that important role in the circle of approach and pollination syndrome for gardeners looking to naturescape. What next stage of parasitism examples to collect or form. For example humans give dogs food building shelter perhaps the dog provides companionship and protection This alongside an. An example relationships. Well and would but to afford more about the process and example support those species. Some examples with needs. Living closer to the sea various, other marine invertebrates such as bivalve mollusks have also established symbioses with chemosynthetic bacteria, where sulfide and example are intermediate in butter water perfusing the sediments. Google map api call the relationship with endophyte fungi because the odours of nutrients to publish articles and examples benefit from a symbiosis? The energy is utilized to synthesize organic molecules from severe carbon dioxide in vent whistle and seawater. Instead, the majority of parasites cause relatively minor except to create host. Scientists have sought to better lock the evolutionary history of bacteria residing within lice In or study will see that bacterial evolution. Microbial parasites with parasitic relationships with the parasitism examples of interaction, lice and plants and meeting are themselves. -
Exploitation: Predation, Herbivory, Parasitism & Disease • Terms
Exploitation: Predation, Herbivory, Parasitism & Disease • Terms Herbivore œ consume plants but usually do not kill them Predator œ kill and consume other organisms Parasites œ live on the tissue of host organisms, usually weakens them but does not usually kill them Parasitoid œ usually kill their host, seen mostly in organisms with rapid life cycles (insects and mites) Pathogens œ induce disease in their hosts 1 Exploitation: Predation, Herbivory, Parasitism & Disease • Parasites and Pathogens That Manipulate Host Behavior œ Parasites That Alter the Behavior of Hosts œ many parasites alter the behavior of the host to spread the parasite further • Acanthocephalans (SpineyHeaded Worms) œ Infect amphipods œ Alter amphipod behavior to make it more likely for them to be ingested by beaver, ducks and muskrats » Uninfected amphipods demonstrate negative phototaxis » Infected organisms demonstrate positive phototaxis œ this brings them closer to the surface of the water and makes them more likely to be eaten 2 Exploitation: Predation, Herbivory, Parasitism & Disease • Janice Moore (1983, 84) œ observed a complex relationship between three organisms: œ An Acanthocephalan, Plagiorhynchus cylindricans œ A terrestrial isopod, a pill bug Armadillidium vulgare, this organism serves as the intermediate host for Plagiorhynchus œ The European Starling, Sturnus vulgaris Initial observations showed that only 1% of pill bugs were infected whereas 40 % of starlings are infected œ from this she proposed that Plagiorhynchus alters the behavior of the pill -
Parasites Shape Community Structure and Dynamics in Freshwater Crustaceans Cambridge.Org/Par
Parasitology Parasites shape community structure and dynamics in freshwater crustaceans cambridge.org/par Olwyn C. Friesen1 , Sarah Goellner2 , Robert Poulin1 and Clément Lagrue1,3 1 2 Research Article Department of Zoology, 340 Great King St, University of Otago, Dunedin 9016, New Zealand; Center for Integrative Infectious Diseases, Im Neuenheimer Feld 344, University of Heidelberg, Heidelberg 69120, Germany 3 Cite this article: Friesen OC, Goellner S, and Department of Biological Sciences, CW 405, Biological Sciences Building, University of Alberta, Edmonton, Poulin R, Lagrue C (2020). Parasites shape Alberta T6G 2E9, Canada community structure and dynamics in freshwater crustaceans. Parasitology 147, Abstract 182–193. https://doi.org/10.1017/ S0031182019001483 Parasites directly and indirectly influence the important interactions among hosts such as competition and predation through modifications of behaviour, reproduction and survival. Received: 24 June 2019 Such impacts can affect local biodiversity, relative abundance of host species and structuring Revised: 27 September 2019 Accepted: 27 September 2019 of communities and ecosystems. Despite having a firm theoretical basis for the potential First published online: 4 November 2019 effects of parasites on ecosystems, there is a scarcity of experimental data to validate these hypotheses, making our inferences about this topic more circumstantial. To quantitatively Key words: test parasites’ role in structuring host communities, we set up a controlled, multigenerational Host community; parasites; population dynamics; species composition mesocosm experiment involving four sympatric freshwater crustacean species that share up to four parasite species. Mesocosms were assigned to either of two different treatments, low or Author for correspondence: high parasite exposure. We found that the trematode Maritrema poulini differentially influ- Olwyn C. -
Surveying for Terrestrial Arthropods (Insects and Relatives) Occurring Within the Kahului Airport Environs, Maui, Hawai‘I: Synthesis Report
Surveying for Terrestrial Arthropods (Insects and Relatives) Occurring within the Kahului Airport Environs, Maui, Hawai‘i: Synthesis Report Prepared by Francis G. Howarth, David J. Preston, and Richard Pyle Honolulu, Hawaii January 2012 Surveying for Terrestrial Arthropods (Insects and Relatives) Occurring within the Kahului Airport Environs, Maui, Hawai‘i: Synthesis Report Francis G. Howarth, David J. Preston, and Richard Pyle Hawaii Biological Survey Bishop Museum Honolulu, Hawai‘i 96817 USA Prepared for EKNA Services Inc. 615 Pi‘ikoi Street, Suite 300 Honolulu, Hawai‘i 96814 and State of Hawaii, Department of Transportation, Airports Division Bishop Museum Technical Report 58 Honolulu, Hawaii January 2012 Bishop Museum Press 1525 Bernice Street Honolulu, Hawai‘i Copyright 2012 Bishop Museum All Rights Reserved Printed in the United States of America ISSN 1085-455X Contribution No. 2012 001 to the Hawaii Biological Survey COVER Adult male Hawaiian long-horned wood-borer, Plagithmysus kahului, on its host plant Chenopodium oahuense. This species is endemic to lowland Maui and was discovered during the arthropod surveys. Photograph by Forest and Kim Starr, Makawao, Maui. Used with permission. Hawaii Biological Report on Monitoring Arthropods within Kahului Airport Environs, Synthesis TABLE OF CONTENTS Table of Contents …………….......................................................……………...........……………..…..….i. Executive Summary …….....................................................…………………...........……………..…..….1 Introduction ..................................................................………………………...........……………..…..….4 -
View the PDF File of the Tachinid Times, Issue 12
The Tachinid Times ISSUE 12 February 1999 Jim O’Hara, editor Agriculture & Agri-Food Canada, Biological Resources Program Eastern Cereal and Oilseed Research Centre C.E.F., Ottawa, Ontario, Canada, K1A 0C6 Correspondence: [email protected] The Tachinid Times began in 1988 when personal Evolution of Egg Structure in Tachinidae (by S.P. computers were gaining in popularity, yet before the Gaponov) advent of e-mail and the World Wide Web. A newsletter Using a scanning electron microscope I investigated distributed through the mail seemed like a useful the egg structure of 114 species of Tachinidae. The endeavour to foster greater awareness about the work of research was focused on the peculiarities of the egg others among researchers interested in the Tachinidae. surface and the structure of the aeropylar area. Data on Now, eleven years later, despite the speed and the method of egg-laying, the structure of the female convenience of e-mail and other advanced modes of reproductive system and the host range were also taken communication, this newsletter still seems to hold a place into consideration. Since any kind of adaptation is a in the distribution of news about the Tachinidae. If there result of evolution and every stage of ontogenesis, is sufficient interest - and submissions - over the course including the egg stage, is adapted to some specific of the next year, then another issue will appear in environmental conditions, each stage of ontogenesis February of the new millennium. As always, please send evolved more or less independently. The development of me your news for inclusion in the newsletter before the provisionary devices (coenogenetic adaptations) and their end of next January. -
Molecular Identification of a Parasitic
& Herpeto gy lo lo gy o : h C it u n r r r e O n , t y R g e o l Entomology, Ornithology & s o e a m r o c t Guerra et al, Entomol Ornithol Herpetol 2014, 3:3 h n E DOI: 10.4172/2161-0983.1000131 ISSN: 2161-0983 Herpetology: Current Research Short Communication Open Access Molecular Identification of a Parasitic Fly (Diptera: Tachinidae) from the Introduced Helicoverpa armigera (Lepidoptera: Noctuidae) in Brazil Guerra WD1, Guerra ALLD2, Ribas LN3, Gonçalves RM4 and Mastrangelo T4* 1Ministry of Agriculture, Livestock and Food Supply, Federal Agricultural Superintendence in Mato Grosso, 78115-000, Brazil 2Federal University of Mato Grosso, 78060-900, Brazil 3Associação dos Produtores de Soja e Milho do Mato Grosso (Aprosoja), 78049-908, Brazil 4 Center for Molecular Biology and Genetic Engineering, State University of Campinas, 13083-875, Brazil *Corresponding author: Thiago Mastrangelo, Center for Molecular Biology and Genetic Engineering, State University of Campinas (UNICAMP), Av. Candido Rondon 400, 13083-875, Campinas, SP, Brazil, Tel: +55 19 3521 1141; E-mail: [email protected] Rec date: June 24, 2014;Acc date: June 26, 2014;Pub date: Jul 13, 2014 Copyright: © 2014 Guerra WD, et al. This is an open-access article distributed under the terms of the Creative Commons Attribution License, which permits unrestricted use, distribution, and reproduction in any medium, provided the original author and source are credited. Abstract The African cotton bollworm, Helicoverpa armigera Hubner (Lepidoptera: Noctuidae) was detected in Brazil, triggering a major phytosanitary crisis during the 2012/2013 harvest. Despite its recent introduction, larvae were found being parasitized by indigenous dipterans with estimated parasitism rates even higher than 30% at some localities. -
Redalyc.Contribution to a History of Mexican Dipterology Part II.- The
Acta Zoológica Mexicana (nueva serie) ISSN: 0065-1737 [email protected] Instituto de Ecología, A.C. México Papavero, Nelson; Ibáñez Bernal, Sergio Contribution to a History of Mexican Dipterology Part II.- The Biologia Centrali-Americana Acta Zoológica Mexicana (nueva serie), núm. 88, 2003, pp. 143 - 232 Instituto de Ecología, A.C. Xalapa, México Available in: http://www.redalyc.org/articulo.oa?id=57508806 How to cite Complete issue Scientific Information System More information about this article Network of Scientific Journals from Latin America, the Caribbean, Spain and Portugal Journal's homepage in redalyc.org Non-profit academic project, developed under the open access initiative Acta Zool. Mex. (n.s.) 88:143-232 (2003) CONTRIBUTIONS TO A HISTORY OF MEXICAN DIPTEROLOGY. PART II.- THE BIOLOGIA CENTRALI-AMERICANA Nelson PAPAVERO1 & Sergio IBÁÑEZ-BERNAL2 1 Museu de Zoologia & Instituto de Estudos Avançados, Universidade de Sao Paulo, Sao Paulo, SP, BRAZIL Pesquisador do Conselho Nacional de Desenvolvimento Científico e Tecnológico (CNPq, Proc. Nº 1 300994/79) 2 Instituto de Ecología, A.C. Departamento de Entomología, km 2.5 carretera antigua a Coatepec N/ 351, Congregación El Haya, 91070, Xalapa, Veracruz, MÉXICO RESUMEN En esta segunda contribución a la historia de la Dipterología mexicana, se presentan datos generales de la obra Biologia-Centrali Americana, de sus autores, colectores y de los viajes efectuados para la obtención del material. Específicamente con respecto a Diptera, se incluyen algunos aspectos de la vida y obra de los contribuidores de este trabajo. Aquí se enlistan todos los nombres de especies de los Diptera mexicanos propuestos por Karl Robert Romanovitch Baron von den Osten Sacken (78 especies), Samuel Wendell Williston (200 especies), John Merton Aldrich (47 especies), William Morton Wheeler y Axel Leonard Melander (18 especies), y Frederik Maurits Van Der Wulp (610 especies).