Proteostasis Perturbation Destabilizes Respiratory Complex Assembly
Total Page:16
File Type:pdf, Size:1020Kb
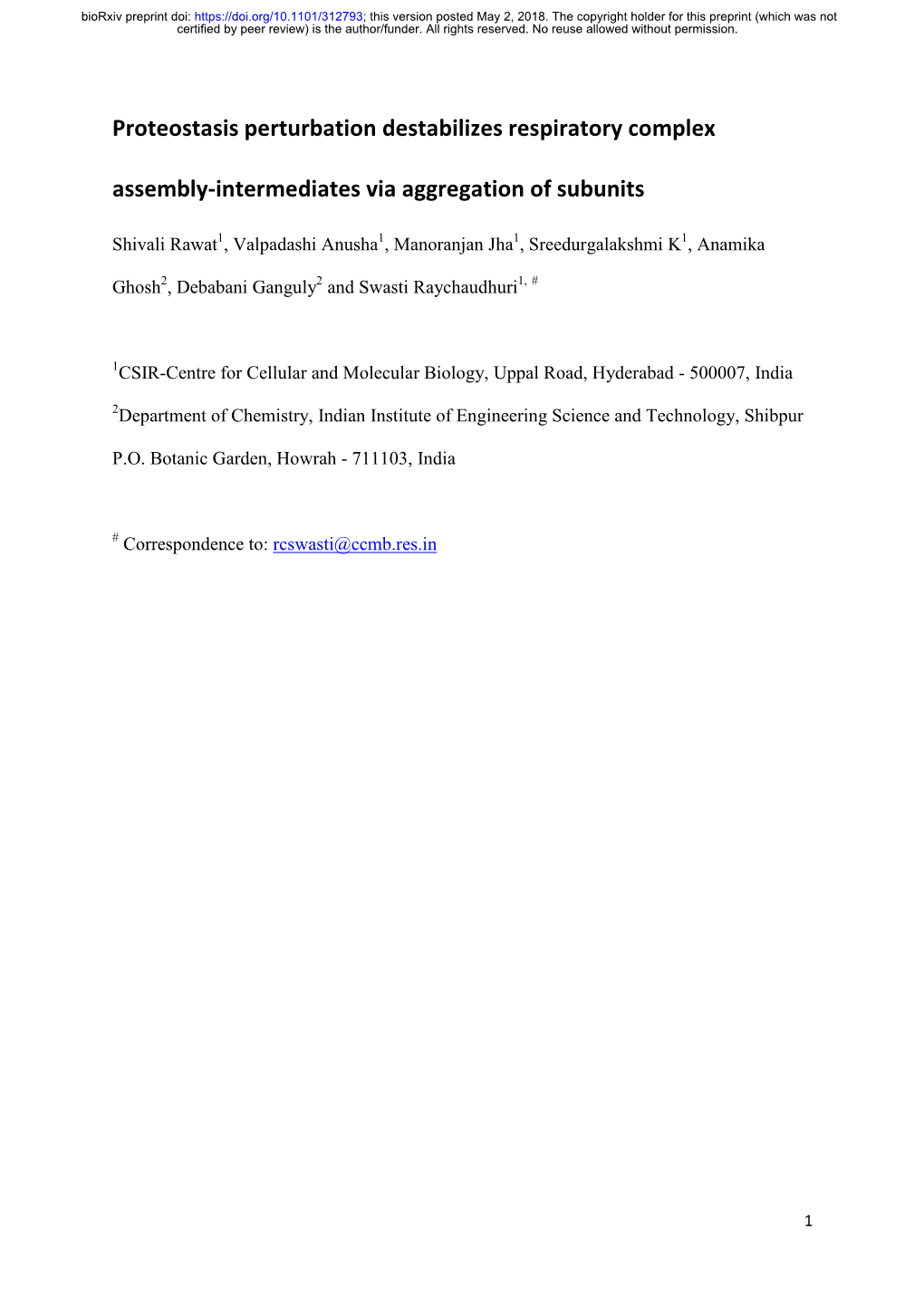
Load more
Recommended publications
-
Increased COUP-TFII Expression in Adult Hearts Induces Mitochondrial Dysfunction Resulting in Heart Failure
ARTICLE Received 9 Mar 2015 | Accepted 30 Jul 2015 | Published 10 Sep 2015 DOI: 10.1038/ncomms9245 OPEN Increased COUP-TFII expression in adult hearts induces mitochondrial dysfunction resulting in heart failure San-Pin Wu1,2, Chung-Yang Kao1, Leiming Wang1, Chad J. Creighton3,4, Jin Yang5, Taraka R. Donti6, Romain Harmancey7, Hernan G. Vasquez7, Brett H. Graham6,8, Hugo J. Bellen6,8, Heinrich Taegtmeyer7, Ching-Pin Chang5, Ming-Jer Tsai1,8 & Sophia Y. Tsai1,8 Mitochondrial dysfunction and metabolic remodelling are pivotal in the development of cardiomyopathy. Here, we show that myocardial COUP-TFII overexpression causes heart failure in mice, suggesting a causal effect of elevated COUP-TFII levels on development of dilated cardiomyopathy. COUP-TFII represses genes critical for mitochondrial electron transport chain enzyme activity, oxidative stress detoxification and mitochondrial dynamics, resulting in increased levels of reactive oxygen species and lower rates of oxygen consumption in mitochondria. COUP-TFII also suppresses the metabolic regulator PGC-1 network and decreases the expression of key glucose and lipid utilization genes, leading to a reduction in both glucose and oleate oxidation in the hearts. These data suggest that COUP- TFII affects mitochondrial function, impairs metabolic remodelling and has a key role in dilated cardiomyopathy. Last, COUP-TFII haploinsufficiency attenuates the progression of cardiac dilation and improves survival in a calcineurin transgenic mouse model, indicating that COUP-TFII may serve as a therapeutic target for the treatment of dilated cardiomyopathy. 1 Department of Molecular and Cellular Biology, Baylor College of Medicine, Houston, Texas 77030, USA. 2 Adrienne Helis Malvin Medical Research Foundation, New Orleans, Louisiana 70130, USA. -
Supplementary Materials: Evaluation of Cytotoxicity and Α-Glucosidase Inhibitory Activity of Amide and Polyamino-Derivatives of Lupane Triterpenoids
Supplementary Materials: Evaluation of cytotoxicity and α-glucosidase inhibitory activity of amide and polyamino-derivatives of lupane triterpenoids Oxana B. Kazakova1*, Gul'nara V. Giniyatullina1, Akhat G. Mustafin1, Denis A. Babkov2, Elena V. Sokolova2, Alexander A. Spasov2* 1Ufa Institute of Chemistry of the Ufa Federal Research Centre of the Russian Academy of Sciences, 71, pr. Oktyabrya, 450054 Ufa, Russian Federation 2Scientific Center for Innovative Drugs, Volgograd State Medical University, Novorossiyskaya st. 39, Volgograd 400087, Russian Federation Correspondence Prof. Dr. Oxana B. Kazakova Ufa Institute of Chemistry of the Ufa Federal Research Centre of the Russian Academy of Sciences 71 Prospeсt Oktyabrya Ufa, 450054 Russian Federation E-mail: [email protected] Prof. Dr. Alexander A. Spasov Scientific Center for Innovative Drugs of the Volgograd State Medical University 39 Novorossiyskaya st. Volgograd, 400087 Russian Federation E-mail: [email protected] Figure S1. 1H and 13C of compound 2. H NH N H O H O H 2 2 Figure S2. 1H and 13C of compound 4. NH2 O H O H CH3 O O H H3C O H 4 3 Figure S3. Anticancer screening data of compound 2 at single dose assay 4 Figure S4. Anticancer screening data of compound 7 at single dose assay 5 Figure S5. Anticancer screening data of compound 8 at single dose assay 6 Figure S6. Anticancer screening data of compound 9 at single dose assay 7 Figure S7. Anticancer screening data of compound 12 at single dose assay 8 Figure S8. Anticancer screening data of compound 13 at single dose assay 9 Figure S9. Anticancer screening data of compound 14 at single dose assay 10 Figure S10. -
Whole-Genome Microarray Detects Deletions and Loss of Heterozygosity of Chromosome 3 Occurring Exclusively in Metastasizing Uveal Melanoma
Anatomy and Pathology Whole-Genome Microarray Detects Deletions and Loss of Heterozygosity of Chromosome 3 Occurring Exclusively in Metastasizing Uveal Melanoma Sarah L. Lake,1 Sarah E. Coupland,1 Azzam F. G. Taktak,2 and Bertil E. Damato3 PURPOSE. To detect deletions and loss of heterozygosity of disease is fatal in 92% of patients within 2 years of diagnosis. chromosome 3 in a rare subset of fatal, disomy 3 uveal mela- Clinical and histopathologic risk factors for UM metastasis noma (UM), undetectable by fluorescence in situ hybridization include large basal tumor diameter (LBD), ciliary body involve- (FISH). ment, epithelioid cytomorphology, extracellular matrix peri- ϩ ETHODS odic acid-Schiff-positive (PAS ) loops, and high mitotic M . Multiplex ligation-dependent probe amplification 3,4 5 (MLPA) with the P027 UM assay was performed on formalin- count. Prescher et al. showed that a nonrandom genetic fixed, paraffin-embedded (FFPE) whole tumor sections from 19 change, monosomy 3, correlates strongly with metastatic death, and the correlation has since been confirmed by several disomy 3 metastasizing UMs. Whole-genome microarray analy- 3,6–10 ses using a single-nucleotide polymorphism microarray (aSNP) groups. Consequently, fluorescence in situ hybridization were performed on frozen tissue samples from four fatal dis- (FISH) detection of chromosome 3 using a centromeric probe omy 3 metastasizing UMs and three disomy 3 tumors with Ͼ5 became routine practice for UM prognostication; however, 5% years’ metastasis-free survival. to 20% of disomy 3 UM patients unexpectedly develop metas- tases.11 Attempts have therefore been made to identify the RESULTS. Two metastasizing UMs that had been classified as minimal region(s) of deletion on chromosome 3.12–15 Despite disomy 3 by FISH analysis of a small tumor sample were found these studies, little progress has been made in defining the key on MLPA analysis to show monosomy 3. -
Nuclear Ubiquitin-Proteasome Pathways in Proteostasis Maintenance
biomolecules Review Nuclear Ubiquitin-Proteasome Pathways in Proteostasis Maintenance Dina Frani´c †, Klara Zubˇci´c † and Mirta Boban * Croatian Institute for Brain Research, School of Medicine, University of Zagreb, 10000 Zagreb, Croatia; [email protected] (D.F.); [email protected] (K.Z.) * Correspondence: [email protected] † Equal contribution. Abstract: Protein homeostasis, or proteostasis, is crucial for the functioning of a cell, as proteins that are mislocalized, present in excessive amounts, or aberrant due to misfolding or other type of damage can be harmful. Proteostasis includes attaining the correct protein structure, localization, and the for- mation of higher order complexes, and well as the appropriate protein concentrations. Consequences of proteostasis imbalance are evident in a range of neurodegenerative diseases characterized by protein misfolding and aggregation, such as Alzheimer’s, Parkinson’s, and amyotrophic lateral sclerosis. To protect the cell from the accumulation of aberrant proteins, a network of protein quality control (PQC) pathways identifies the substrates and direct them towards refolding or elimination via regulated protein degradation. The main pathway for degradation of misfolded proteins is the ubiquitin-proteasome system. PQC pathways have been first described in the cytoplasm and the endoplasmic reticulum, however, accumulating evidence indicates that the nucleus is an important PQC compartment for ubiquitination and proteasomal degradation of not only nuclear, but also cyto- plasmic proteins. In this review, we summarize the nuclear ubiquitin-proteasome pathways involved in proteostasis maintenance in yeast, focusing on inner nuclear membrane-associated degradation (INMAD) and San1-mediated protein quality control. Keywords: proteasome; ubiquitin; nucleus; inner nuclear membrane; yeast; proteostasis; protein quality control; protein misfolding Citation: Frani´c,D.; Zubˇci´c,K.; Boban, M. -
Assembly Factors for the Membrane Arm of Human Complex I
Assembly factors for the membrane arm of human complex I Byron Andrews, Joe Carroll, Shujing Ding, Ian M. Fearnley, and John E. Walker1 Medical Research Council Mitochondrial Biology Unit, Cambridge CB2 0XY, United Kingdom Contributed by John E. Walker, October 14, 2013 (sent for review September 12, 2013) Mitochondrial respiratory complex I is a product of both the nuclear subunits in a fungal enzyme from Yarrowia lipolytica seem to be and mitochondrial genomes. The integration of seven subunits distributed similarly (12, 13). encoded in mitochondrial DNA into the inner membrane, their asso- The assembly of mitochondrial complex I involves building the ciation with 14 nuclear-encoded membrane subunits, the construc- 44 subunits emanating from two genomes into the two domains of tion of the extrinsic arm from 23 additional nuclear-encoded the complex. The enzyme is put together from preassembled sub- proteins, iron–sulfur clusters, and flavin mononucleotide cofactor complexes, and their subunit compositions have been characterized require the participation of assembly factors. Some are intrinsic to partially (14, 15). Extrinsic assembly factors of unknown function the complex, whereas others participate transiently. The suppres- become associated with subcomplexes that accumulate when as- sion of the expression of the NDUFA11 subunit of complex I dis- sembly and the activity of complex I are impaired by pathogenic rupted the assembly of the complex, and subcomplexes with mutations. Some assembly factor mutations also impair its activ- masses of 550 and 815 kDa accumulated. Eight of the known ex- ity (16). Other pathogenic mutations are found in all of the core trinsic assembly factors plus a hydrophobic protein, C3orf1, were subunits, and in 10 supernumerary subunits (NDUFA1, NDUFA2, associated with the subcomplexes. -
NDUFS6 Mutations Are a Novel Cause of Lethal Neonatal Mitochondrial Complex I Deficiency Denise M
Related Commentary, page 760 Research article NDUFS6 mutations are a novel cause of lethal neonatal mitochondrial complex I deficiency Denise M. Kirby,1,2,3 Renato Salemi,1 Canny Sugiana,1,3 Akira Ohtake,4 Lee Parry,1 Katrina M. Bell,1 Edwin P. Kirk,5 Avihu Boneh,1,2,3 Robert W. Taylor,6 Hans-Henrik M. Dahl,1,3 Michael T. Ryan,4 and David R. Thorburn1,2,3 1Murdoch Childrens Research Institute and 2Genetic Health Services Victoria, Royal Children’s Hospital, Melbourne, Victoria, Australia. 3Department of Paediatrics, University of Melbourne, Melbourne, Victoria, Australia. 4Department of Biochemistry, LaTrobe University, Melbourne, Victoria, Australia. 5Department of Medical Genetics, Sydney Children’s Hospital, Sydney, New South Wales, Australia. 6Mitochondrial Research Group, School of Neurology, Neurobiology and Psychiatry, University of Newcastle upon Tyne, Newcastle upon Tyne, United Kingdom. Complex I deficiency, the most common respiratory chain defect, is genetically heterogeneous: mutations in 8 nuclear and 7 mitochondrial DNA genes encoding complex I subunits have been described. However, these genes account for disease in only a minority of complex I–deficient patients. We investigated whether there may be an unknown common gene by performing functional complementation analysis of cell lines from 10 unrelated patients. Two of the patients were found to have mitochondrial DNA mutations. The other 8 repre- sented 7 different (nuclear) complementation groups, all but 1 of which showed abnormalities of complex I assembly. It is thus unlikely that any one unknown gene accounts for a large proportion of complex I cases. The 2 patients sharing a nuclear complementation group had a similar abnormal complex I assembly profile and were studied further by homozygosity mapping, chromosome transfers, and microarray expression analysis. -
Molecular Chaperones and Proteolytic Machineries Regulate Protein Homeostasis in Aging Cells
cells Review Molecular Chaperones and Proteolytic Machineries Regulate Protein Homeostasis in Aging Cells Boris Margulis, Anna Tsimokha, Svetlana Zubova and Irina Guzhova * Institute of Cytology of the Russian Academy of Sciences, St. Petersburg 194064, Russia; [email protected] (B.M.); [email protected] (A.T.); [email protected] (S.Z.) * Correspondence: [email protected]; Tel.: +7-812-2973794 Received: 14 April 2020; Accepted: 19 May 2020; Published: 24 May 2020 Abstract: Throughout their life cycles, cells are subject to a variety of stresses that lead to a compromise between cell death and survival. Survival is partially provided by the cell proteostasis network, which consists of molecular chaperones, a ubiquitin-proteasome system of degradation and autophagy. The cooperation of these systems impacts the correct function of protein synthesis/modification/transport machinery starting from the adaption of nascent polypeptides to cellular overcrowding until the utilization of damaged or needless proteins. Eventually, aging cells, in parallel to the accumulation of flawed proteins, gradually lose their proteostasis mechanisms, and this loss leads to the degeneration of large cellular masses and to number of age-associated pathologies and ultimately death. In this review, we describe the function of proteostasis mechanisms with an emphasis on the possible associations between them. Keywords: molecular chaperones; autophagy; ubiquitin-proteasomal system; aging 1. Introduction Aging is a process that, according to Maurice Chevalier, “isn’t so bad when you consider the alternative.” In numerous essays, aging or senescence is presented as a gradual dying process that involves a loss of cell integrity, leading to the dysfunction of cells or organs and eventually to their deaths. -
S41467-020-18249-3.Pdf
ARTICLE https://doi.org/10.1038/s41467-020-18249-3 OPEN Pharmacologically reversible zonation-dependent endothelial cell transcriptomic changes with neurodegenerative disease associations in the aged brain Lei Zhao1,2,17, Zhongqi Li 1,2,17, Joaquim S. L. Vong2,3,17, Xinyi Chen1,2, Hei-Ming Lai1,2,4,5,6, Leo Y. C. Yan1,2, Junzhe Huang1,2, Samuel K. H. Sy1,2,7, Xiaoyu Tian 8, Yu Huang 8, Ho Yin Edwin Chan5,9, Hon-Cheong So6,8, ✉ ✉ Wai-Lung Ng 10, Yamei Tang11, Wei-Jye Lin12,13, Vincent C. T. Mok1,5,6,14,15 &HoKo 1,2,4,5,6,8,14,16 1234567890():,; The molecular signatures of cells in the brain have been revealed in unprecedented detail, yet the ageing-associated genome-wide expression changes that may contribute to neurovas- cular dysfunction in neurodegenerative diseases remain elusive. Here, we report zonation- dependent transcriptomic changes in aged mouse brain endothelial cells (ECs), which pro- minently implicate altered immune/cytokine signaling in ECs of all vascular segments, and functional changes impacting the blood–brain barrier (BBB) and glucose/energy metabolism especially in capillary ECs (capECs). An overrepresentation of Alzheimer disease (AD) GWAS genes is evident among the human orthologs of the differentially expressed genes of aged capECs, while comparative analysis revealed a subset of concordantly downregulated, functionally important genes in human AD brains. Treatment with exenatide, a glucagon-like peptide-1 receptor agonist, strongly reverses aged mouse brain EC transcriptomic changes and BBB leakage, with associated attenuation of microglial priming. We thus revealed tran- scriptomic alterations underlying brain EC ageing that are complex yet pharmacologically reversible. -
Low Abundance of the Matrix Arm of Complex I in Mitochondria Predicts Longevity in Mice
ARTICLE Received 24 Jan 2014 | Accepted 9 Apr 2014 | Published 12 May 2014 DOI: 10.1038/ncomms4837 OPEN Low abundance of the matrix arm of complex I in mitochondria predicts longevity in mice Satomi Miwa1, Howsun Jow2, Karen Baty3, Amy Johnson1, Rafal Czapiewski1, Gabriele Saretzki1, Achim Treumann3 & Thomas von Zglinicki1 Mitochondrial function is an important determinant of the ageing process; however, the mitochondrial properties that enable longevity are not well understood. Here we show that optimal assembly of mitochondrial complex I predicts longevity in mice. Using an unbiased high-coverage high-confidence approach, we demonstrate that electron transport chain proteins, especially the matrix arm subunits of complex I, are decreased in young long-living mice, which is associated with improved complex I assembly, higher complex I-linked state 3 oxygen consumption rates and decreased superoxide production, whereas the opposite is seen in old mice. Disruption of complex I assembly reduces oxidative metabolism with concomitant increase in mitochondrial superoxide production. This is rescued by knockdown of the mitochondrial chaperone, prohibitin. Disrupted complex I assembly causes premature senescence in primary cells. We propose that lower abundance of free catalytic complex I components supports complex I assembly, efficacy of substrate utilization and minimal ROS production, enabling enhanced longevity. 1 Institute for Ageing and Health, Newcastle University, Newcastle upon Tyne NE4 5PL, UK. 2 Centre for Integrated Systems Biology of Ageing and Nutrition, Newcastle University, Newcastle upon Tyne NE4 5PL, UK. 3 Newcastle University Protein and Proteome Analysis, Devonshire Building, Devonshire Terrace, Newcastle upon Tyne NE1 7RU, UK. Correspondence and requests for materials should be addressed to T.v.Z. -
Mitoxplorer, a Visual Data Mining Platform To
mitoXplorer, a visual data mining platform to systematically analyze and visualize mitochondrial expression dynamics and mutations Annie Yim, Prasanna Koti, Adrien Bonnard, Fabio Marchiano, Milena Dürrbaum, Cecilia Garcia-Perez, José Villaveces, Salma Gamal, Giovanni Cardone, Fabiana Perocchi, et al. To cite this version: Annie Yim, Prasanna Koti, Adrien Bonnard, Fabio Marchiano, Milena Dürrbaum, et al.. mitoXplorer, a visual data mining platform to systematically analyze and visualize mitochondrial expression dy- namics and mutations. Nucleic Acids Research, Oxford University Press, 2020, 10.1093/nar/gkz1128. hal-02394433 HAL Id: hal-02394433 https://hal-amu.archives-ouvertes.fr/hal-02394433 Submitted on 4 Dec 2019 HAL is a multi-disciplinary open access L’archive ouverte pluridisciplinaire HAL, est archive for the deposit and dissemination of sci- destinée au dépôt et à la diffusion de documents entific research documents, whether they are pub- scientifiques de niveau recherche, publiés ou non, lished or not. The documents may come from émanant des établissements d’enseignement et de teaching and research institutions in France or recherche français ou étrangers, des laboratoires abroad, or from public or private research centers. publics ou privés. Distributed under a Creative Commons Attribution| 4.0 International License Nucleic Acids Research, 2019 1 doi: 10.1093/nar/gkz1128 Downloaded from https://academic.oup.com/nar/advance-article-abstract/doi/10.1093/nar/gkz1128/5651332 by Bibliothèque de l'université la Méditerranée user on 04 December 2019 mitoXplorer, a visual data mining platform to systematically analyze and visualize mitochondrial expression dynamics and mutations Annie Yim1,†, Prasanna Koti1,†, Adrien Bonnard2, Fabio Marchiano3, Milena Durrbaum¨ 1, Cecilia Garcia-Perez4, Jose Villaveces1, Salma Gamal1, Giovanni Cardone1, Fabiana Perocchi4, Zuzana Storchova1,5 and Bianca H. -
The LYR Protein Subunit NB4M/NDUFA6 of Mitochondrial Complex I Anchors an Acyl Carrier Protein and Is Essential for Catalytic Activity
The LYR protein subunit NB4M/NDUFA6 of mitochondrial complex I anchors an acyl carrier protein and is essential for catalytic activity Heike Angerera, Michael Radermacherb, Michalina Mankowska a, Mirco Stegerc, Klaus Zwickerd, Heinrich Heidec, Ilka Wittigc,e, Ulrich Brandte,f, and Volker Zickermanna,e,1 aInstitute of Biochemistry II, Structural Bioenergetics Group, Medical School, Goethe University Frankfurt, 60438 Frankfurt, Germany; bDepartment of Molecular Physiology and Biophysics, College of Medicine, University of Vermont, Burlington, VT 05405; cFunctional Proteomics, SFB 815 Core Unit, and dInstitute of Biochemistry I, Medical School, Goethe University Frankfurt, 60590 Frankfurt, Germany; eCluster of Excellence Macromolecular Complexes, Goethe University Frankfurt, Germany; and fNijmegen Centre for Mitochondrial Disorders, Radboud University Medical Centre, 6525 GA, Nijmegen, The Netherlands Edited by Wolfgang Baumeister, Max Planck Institute of Biochemistry, Martinsried, Germany, and approved February 25, 2014 (received for review December 4, 2013) Mitochondrial complex I is the largest and most complicated enzyme is identical to LYR motif containing protein 6 (LYRM6). Sever- of the oxidative phosphorylation system. It comprises a number al other LYRM proteins were shown to be associated with of so-called accessory subunits of largely unknown structure and the maintenance of mitochondrial homeostasis. LYRM4 (alterna- function. Here we studied subunit NB4M [NDUFA6, LYR motif tive designation ISD11, iron-sulfur protein biogenesis -
Membrane Proteomics of Cervical Cancer Cell Lines Reveal Insights on the Process of Cervical Carcinogenesis
INTERNATIONAL JOURNAL OF ONCOLOGY 53: 2111-2122, 2018 Membrane proteomics of cervical cancer cell lines reveal insights on the process of cervical carcinogenesis KALLIOPI I. PAPPA1,2, POLYXENI CHRISTOU3,4, AMARILDO XHOLI3, GEORGE MERMELEKAS3, GEORGIA KONTOSTATHI3,4, VASILIKI LYGIROU3,4, MANOUSOS MAKRIDAKIS3, JEROME ZOIDAKIS3 and NICHOLAS P. ANAGNOU1,4 1Cell and Gene Therapy Laboratory, Centre of Basic Research II, Biomedical Research Foundation of the Academy of Athens, 11527 Athens; 2First Department of Obstetrics and Gynecology, University of Athens School of Medicine, Alexandra Hospital, 11528 Athens; 3Biotechnology Division, Centre of Basic Research, Biomedical Research Foundation of the Academy of Athens; 4Laboratory of Biology, University of Athens School of Medicine, 11527 Athens, Greece Received March 22, 2018; Accepted May 4, 2018 DOI: 10.3892/ijo.2018.4518 Abstract. The available therapeutic approaches for cervical biological pathways relevant to malignancy, including ‘HIPPO cancer can seriously affect the fertility potential of patient; signaling’, ‘PI3K/Akt signaling’, ‘cell cycle: G2/M DNA thus, there is a pressing requirement for less toxic and damage checkpoint regulation’ and ‘EIF2 signaling’. These targeted therapies. The membrane proteome is a potential unique membrane protein identifications offer insights on a source of therapeutic targets; however, despite the signifi- previously inaccessible region of the cervical cancer proteome, cance of membrane proteins in cancer, proteomic analysis and may represent putative