The Basolateral Amygdala and Superior Colliculus Regulate Physiological and Behavioral Measures of Avoidance and Approach
Total Page:16
File Type:pdf, Size:1020Kb
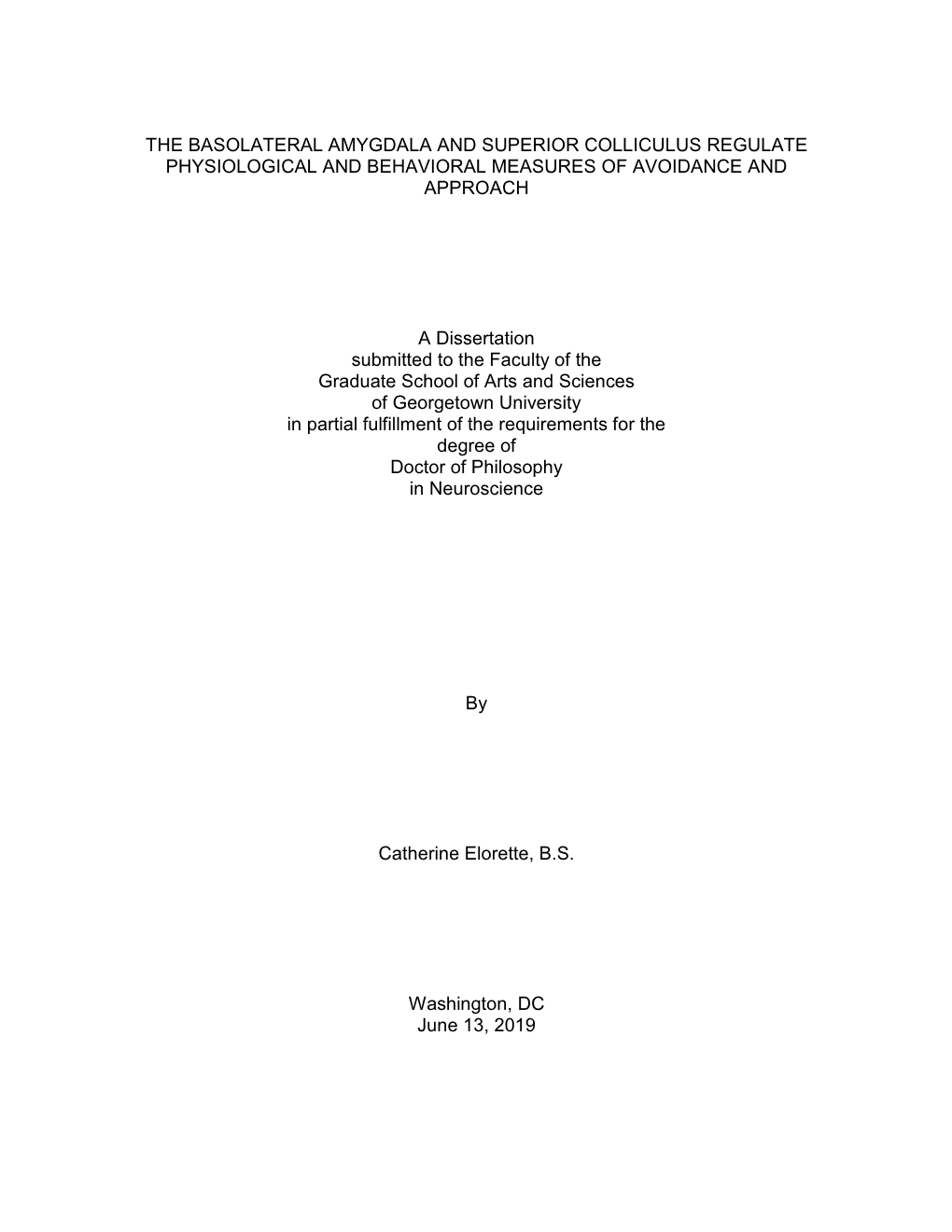
Load more
Recommended publications
-
A Cyclops and a Synotus by K
J Neurol Psychopathol: first published as 10.1136/jnnp.s1-17.65.48 on 1 July 1936. Downloaded from 48 ORIGINAL PAPERS A CYCLOPS AND A SYNOTUS BY K. H. BOUMAN, AMSTERDAM, AND V. W. D. SCHENK, TiH HAGUE INTRODUCTION ONLY a small number of cases of cyclopia in human beings and mammals have been minutely examined. The number becomes still smaller if a more or less complete microscopic investigation of the central nervous system is stipulated. It is really only the cases of Davidson Black and Winkler and perhaps that of Naegli which answer this requirement. In contrast therewith there is an abundance of experimental studies in this field in urodela and other lower classes of animals. For all that, unanimity does not by any means prevail here, although the Protected by copyright. views of Stockard and his followers-who held that the first determination of the eye lay unpaired in the median line-and those of Spemann-who pointed to a paired rudiment from the outset, which views were originally diametrically opposed-appear to have drawn somewhat nearer to each other in recent years. Woerdeman, for instance, found that the paired rudiment of the eye shifts its position laterally downwards very early (when the folds of the medullary plate become visible) and he rightly says that this is not the same as Stockard's lateral growth of an unpaired eye rudiment. Yet, by saying this, he admits certain changes and growth conditions to which Fischel, for instance, did not do full justice. E. Manchot, on the other hand, who defends Stockard's views, admits that between the two regions of the eye http://jnnp.bmj.com/ rudiment there must be a tract of brain tissue (lamina terminalis and regio chiasmatica). -
Hamburger Hamilton Stages
UNSW Embryology- Chicken Development Stages http://embryology.med.unsw.edu.au/OtherEmb/chick1.htm Hamburger Hamilton Stages Hamburger Identification of Hamilton Age Stages Stages Before Laying Shell membrane of 3.5-4.5 Early egg formed in hr cleavage isthmus of oviduct Germ wall formed During from marginal cleavage periblast 4.5-24.0 Shell of egg Late cleavage hr formed in uterus After Laying Preprimitive streak 1 (embryonic shield) Initial primitive 2 6-7 hr streak, 0.3-0.5 mm long Intermediate 12-13 hr 3 primitive streak Definitive primitive 4 18-19 hr streak, ±1.88 mm long Head process 19-22 hr 5 (notochord) 6 23-25 hr Head fold 1 somite; neural 23-26 hr 7 folds ca. 1-3 somites; 7 to 8- 23-26 hr coelom 1 / 5 2007/03/20 9:05 UNSW Embryology- Chicken Development Stages http://embryology.med.unsw.edu.au/OtherEmb/chick1.htm 4 somites; blood 26-29 hr 8 islands 7 somites; primary 29-33 hr 9 optic vesicles 8-9 somites; 9+ to 10- ca. 33 hr anterior amniotic fold 10 somites; 3 10 33-38 hr primary brain vesicles 13 somites; 5 11 40-45 hr neuromeres of hindbrain 16 somites; 45-49 hr 12 telencephalon 19 somites; 13 48-52 hr atrioventricular canal ca. 20-21 somites; tail 13+ to 14- 50-52 hr bud 22 somites; trunk flexure; visceral 50-53 hr 14 arches I and II, clefts 1 and 2 23 somites; ca. premandibular 14+ to 15- 50-54 hr head cavities 24-27 somites; 15 50-55 hr visceral arch III, cleft 3 26-28 somites; 16 51-56 hr wing bud; posterior 2 / 5 2007/03/20 9:05 UNSW Embryology- Chicken Development Stages http://embryology.med.unsw.edu.au/OtherEmb/chick1.htm -
Nervous System Cns
THE NERVOUS SYSTEM CNS • Function The Spinal Cord • General Structure • Enclosed In: • Neural Foramen – length • Connects With: • Foramen magnum – need for protection Coverings • Coverings: • meninges (3) • Subarachnoid space • location • composition • diagnostic use Spinal Nerves • Caudal equina Finer Structures of Spinal Cord • Gray Matter • composition • function • horns (2) • horns form roots (2) • gray commisure • central canal Finer Structures of Spinal Cord • White matter • arrangement • columns contain tracts • description • names The Brain •General Structure •Protection •Skeletal •Membranous The Brain • Development • Neural Plate • Neural Tube and 3 swellings The Brain • Other Structures • Ventricles • Foramen of Monroe • Cerebral Aqueduct The Brain - finer structures • Brain Stem • Medulla • location • connection • gray matter vs. white matter • function • kinds of reflexes The Brain • Brain stem • Pons • Structure • Composition • Nerves The Brain • Brain stem • Midbrain • Location • Composition: • Cerebral peduncles • Substantia nigra • Tegmentum • Corpora quadrigemina • Cerebral aqueduct The Brain • Cerebellum • Location • Structure • Cortex The Brain • Cerebellum • White Matter • Cerebellar Nuclei • Dentate Nuclei • Furrows • Divisions • Functions The Brain • Interbrain • Contains structures (2) • Location • How functions were determined Interbrain • The Thalamus • Function • Result of Injury Interbrain • The Hypothalmus • Function • Reason for these functions • Result of Injury The Brain • The Cerebrum • Size • Complexity • -
Metabolic Responses in Discrete, Mice Brain Regions Like CC, CG, H and CQ During PTZ Induced Epileptic Seizures
Current Neurobiology 2012; 3 (1): 31-38 ISSN 0975-9042 Scientific Publishers of India Metabolic responses in discrete, Mice brain regions like CC, CG, H and CQ during PTZ induced epileptic seizures. K. K. Therisa and P.V. Desai Department of Zoology, Goa University, Taleigao Plateau, Goa 403206, India. Abstract Epilepsy, a neurological disorder with recurrent seizures, involves disruption of different metabolic enzymes and its related metabolites altering the normal processes of metabolism, either during the onset or post epilepsy in the brain. In the present work, it is convincingly, observed that the Mice brain regions such as Corpus callosum (CC), Cingulate gyrus (CG), Hippocampus (H) and Corpora quadrigemina (CQ) shows significant changes in the activi- ties of metabolic enzymes such as AST, ALT, LDH; ATPases like Na +, K +-ATPase, Mg 2+ - ATPase, Ca 2+ -ATPase along with their metabolites such as Glucose, Pyruvate, Lactate and Glutamate, altering the metabolic integrity during Pentylenetetrazole (PTZ) induced epilep- tic seizure. We report from the present work that, H and CG are affected completely during epileptic seizure as compared to its control but CC and CQ shows partly altered as far as metabolism in brain is concerned. Keywords: Corpus callosum, Hippocampus, Cingulate gyrus, Corpora Quadrigemina, Metabolic enzymes and metabo- lites. Accepted April 07 2012 Introduction may overlap with other frontal lobe epilepsy syndromes. But, an aberrant behaviors observed in epileptic patients Epilepsy, involves a disruption of brain energy homeosta- completely resolved after lesionectomy of Cingulate sis and is potentially manageable through principles of gyrus [6]. Hippocampus and Cingulate gyrus forms the metabolic control theory. -
The Brain and Behavior
This page intentionally left blank The Brain and Behavior The Brain and Behavior An Introduction to Behavioral Neuroanatomy Third Edition David L. Clark Nash N. Boutros Mario F. Mendez CAMBRIDGE UNIVERSITY PRESS Cambridge, New York, Melbourne, Madrid, Cape Town, Singapore, São Paulo, Delhi, Dubai, Tokyo Cambridge University Press The Edinburgh Building, Cambridge CB2 8RU, UK Published in the United States of America by Cambridge University Press, New York www.cambridge.org Information on this title: www.cambridge.org/9780521142298 © D. Clark, N. Boutros, M. Mendez 2010 This publication is in copyright. Subject to statutory exception and to the provision of relevant collective licensing agreements, no reproduction of any part may take place without the written permission of Cambridge University Press. First published in print format 2010 ISBN-13 978-0-511-77469-0 eBook (EBL) ISBN-13 978-0-521-14229-8 Paperback Cambridge University Press has no responsibility for the persistence or accuracy of urls for external or third-party internet websites referred to in this publication, and does not guarantee that any content on such websites is, or will remain, accurate or appropriate. Every effort has been made in preparing this book to provide accurate and up-to- date information which is in accord with accepted standards and practice at the time of publication. Although case histories are drawn from actual cases, every effort has been made to disguise the identities of the individuals involved. Nevertheless, the authors, editors, and publishers can make no warranties that the information contained herein is totally free from error, not least because clinical standards are constantly changing through research and regulation. -
Chapter 3: Internal Anatomy of the Central Nervous System
10353-03_CH03.qxd 8/30/07 1:12 PM Page 82 3 Internal Anatomy of the Central Nervous System LEARNING OBJECTIVES Nuclear structures and fiber tracts related to various functional systems exist side by side at each level of the After studying this chapter, students should be able to: nervous system. Because disease processes in the brain • Identify the shapes of corticospinal fibers at different rarely strike only one anatomic structure or pathway, there neuraxial levels is a tendency for a series of related and unrelated clinical symptoms to emerge after a brain injury. A thorough knowl- • Recognize the ventricular cavity at various neuroaxial edge of the internal brain structures, including their shape, levels size, location, and proximity, makes it easier to understand • Recognize major internal anatomic structures of the their functional significance. In addition, the proximity of spinal cord and describe their functions nuclear structures and fiber tracts explains multiple symp- toms that may develop from a single lesion site. • Recognize important internal anatomic structures of the medulla and explain their functions • Recognize important internal anatomic structures of the ANATOMIC ORIENTATION pons and describe their functions LANDMARKS • Identify important internal anatomic structures of the midbrain and discuss their functions Two distinct anatomic landmarks used for visual orientation to the internal anatomy of the brain are the shapes of the • Recognize important internal anatomic structures of the descending corticospinal fibers and the ventricular cavity forebrain (diencephalon, basal ganglia, and limbic (Fig. 3-1). Both are present throughout the brain, although structures) and describe their functions their shape and size vary as one progresses caudally from the • Follow the continuation of major anatomic structures rostral forebrain (telencephalon) to the caudal brainstem. -
Brain Anatomy
BRAIN ANATOMY Adapted from Human Anatomy & Physiology by Marieb and Hoehn (9th ed.) The anatomy of the brain is often discussed in terms of either the embryonic scheme or the medical scheme. The embryonic scheme focuses on developmental pathways and names regions based on embryonic origins. The medical scheme focuses on the layout of the adult brain and names regions based on location and functionality. For this laboratory, we will consider the brain in terms of the medical scheme (Figure 1): Figure 1: General anatomy of the human brain Marieb & Hoehn (Human Anatomy and Physiology, 9th ed.) – Figure 12.2 CEREBRUM: Divided into two hemispheres, the cerebrum is the largest region of the human brain – the two hemispheres together account for ~ 85% of total brain mass. The cerebrum forms the superior part of the brain, covering and obscuring the diencephalon and brain stem similar to the way a mushroom cap covers the top of its stalk. Elevated ridges of tissue, called gyri (singular: gyrus), separated by shallow groves called sulci (singular: sulcus) mark nearly the entire surface of the cerebral hemispheres. Deeper groves, called fissures, separate large regions of the brain. Much of the cerebrum is involved in the processing of somatic sensory and motor information as well as all conscious thoughts and intellectual functions. The outer cortex of the cerebrum is composed of gray matter – billions of neuron cell bodies and unmyelinated axons arranged in six discrete layers. Although only 2 – 4 mm thick, this region accounts for ~ 40% of total brain mass. The inner region is composed of white matter – tracts of myelinated axons. -
BRAINSTEM,CEREBELLUM, DIENCEPHALON-Dr. Kibe INTRODUCTION the Brain Is One of the Largest Organs in Adults
THE CENTRAL NERVOUS SYSTEM BRAINSTEM,CEREBELLUM, DIENCEPHALON-Dr. Kibe INTRODUCTION The brain is one of the largest organs in adults. It consists approximately 100 billion neurons and 900 billion glia . And it weighs about 1.4 kg in adults Neurons of the brain undergo mitotic cell division only during the prenatal period and the first few months of postnatal life. No increase in number after that. Malnutrition during the crucial prenatal months of neuron multiplication is reported to hinder the process and result in fewer brain cells. The brain attains full size by about the eighteenth year but grows rapidly only during the first 9 years or so. BRAINSTEM • Three divisions of the brain make up the brainstem . • The medulla oblongata forms the lowest part of the brainstem, • The midbrain forms the uppermost part, • The pons lies between them, that is, above the medulla and below the midbrain. BRAIN STEM AND DIENCEPHALON Medulla Oblongata • The medulla oblongata is the part of the brain that attaches to the spinal cord. • It measures only a few centimeters (about 1 inch) in length and is separated from the pons above by a horizontal groove. • It is composed of white matter and a network of gray and white matter called the reticular formation • The pyramids are two bulges of white matter located on the ventral surface of the medulla. Fibers of the so-called pyramidal tracts form the pyramids. • The olive of the medulla is an oval projection appearing one on each side of the ventral surface of the medulla, lateral to the pyramids. -
Brain Stem Consists: - Medulla Oblongata - Pons - Midbrain (Mesencephalon)
Brain Stem Consists: - Medulla oblongata - Pons - Midbrain (Mesencephalon) Lies upon the basal portion of occipital bone (clivus) and is connected to cerebellum rostral : diencephalon caudal : spinal cord Contains numerous ascending and descending fibre tracts Brain stem nuclei receive fibres from or sent fibres into cranial nerves (III-XII) attach to the surface of the brain stem cranial nerve nuclei Ascenden and Descenden Pathway of Brain Stem Ascenden Descenden Lemniscus medialis Traktus corticospinalis Tractus spinothalamicus Tractus corticonuclearis Lemniscus trigeminalis Corticopontine fibres Lemniscus lateralis Tractus rubrospinalis Reticularis fibres system Tractus tectospinalis Fasciculus longitudinalis medialis Fasciculuc longitudinal medialis Pedunculus cerebellaris superior Tractus vestibulospinalis Pedunculus cerebellaris inferior Tractus reticulospinalis Secondary vestibularis fibres Tractus tegmentalis centralis Secondary gustatorius fibres Tractus descenden N.V Brain Stem Contains a complex and heterogeneous matrix of neurones reticular formation functions : - control over the level of consciousness - the perception of pain - regulation of the cardiovascular and respiratory systems It also has extensive connections with cranial nerve nuclei, cerebellum, brain stem and spinal motor mechanisms movement, posture and muscle tone Dorsal Surface (External Feature) Peduncles Dorsal median sulcus Dorsal columns (fasciculi gracilis and cuneatus) Gracile and cuneate tubercles (nuclei gracilis and cuneatus) Fossa rhomboidea -
Histopathology IMPC HIS 003
Histopathology IMPC_HIS_003 Purpose To perform histopathological examination and annotation of the IMPC’s standard list of organs (see IMPReSS Gross Pathology & Tissue Collection) on stained tissue sections oriented on glass slides (see IMPReSS Tissue Embedding & Block Banking). Experimental Design Minimum number of animals : 2M + 2F Age at test: Week 16 Notes Significance Score Not significant . Interpreted by the histopathologist to be a finding attributable to background strain (e.g. low- incidence hydrocephalus, microphthalmia) or incidental to mutant phenotype (e.g. hair- induced glossitis, focal hyperplasia, mild mononuclear cell infiltrate). OR Significant . Interpreted by the histopathologist as a finding not attributable to background strain and not incidental to mutant phenotype. Severity Score Sc Term Definition ore No lesion(s) or abnormalities detectable considering the age and sex of 0 Normal the animal Abnormality visible, involving single or multiple tissue types in a minimal 1 Mild proportion of an organ, likely to have no functional consequence 2 Moder Abnormality visible, involving multiple tissue types in a minority proportion ate of an organ, likely to have no clinical consequence (subclinical) Abnormality clearly visible, involving multiple tissue types in a majority 3 Marked proportion of an organ, likely to have minor clinical manifestation(s) Abnormality clearly visible, involving multiple tissue types in almost all 4 Severe visible area of an organ, likely to have major clinical manifestation(s) Parameters and Metadata Adrenal gland - Descriptor PATO IMPC_HIS_107_001 | v1.0 ontologyParameter Req. Analysis: false Req. Upload: false Is Annotated: false Ontology Options: acute, right, left, subacute, random pattern, multifocal to coalescing, multi-localised, generalized, segmental, distributed, chronic-active, chronic, focally extensive, localized, transmural, bilateral, unilateral, symmetrical, peracute, Adrenal gland - MA term IMPC_HIS_350_001 | v1.0 ontologyParameter Req. -
Brainstem: Midbrain
Brainstem: Midbrain 1. Midbrain – gross external anatomy 2. Internal structure of the midbrain: cerebral peduncles tegmentum tectum (guadrigeminal plate) Midbrain Midbrain – general features location – between forebrain and hindbrain the smallest region of the brainstem – 6-7g the shortest brainstem segment ~ 2 cm long least differentiated brainstem division human midbrain is archipallian – shared general architecture with the most ancient of vertebrates embryonic origin – mesencephalon main functions: a sort of relay station for sound and visual information serves as a nerve pathway of the cerebral hemispheres controls the eye movement involved in control of body movement Prof. Dr. Nikolai Lazarov 2 Midbrain Midbrain – gross anatomy dorsal part – tectum (quadrigeminal plate): superior colliculi inferior colliculi cerebral aqueduct ventral part – cerebral peduncles: dorsal – tegmentum (central part) ventral – cerebral crus substantia nigra Prof. Dr. Nikolai Lazarov 3 Midbrain Cerebral crus – internal structure Cerebral peduncle: crus cerebri tegmentum mesencephali substantia nigra two thick semilunar white matter bundles composition – somatotopically arranged motor tracts: corticospinal } pyramidal tracts – medial ⅔ corticobulbar corticopontine fibers: frontopontine tracts – medially temporopontine tracts – laterally interpeduncular fossa (of Tarin ) posterior perforated substance Prof. Dr. Nikolai Lazarov 4 Midbrain Midbrain tegmentum – internal structure crus cerebri tegmentum mesencephali substantia -
The Brain, Cranial Nerves, and Sensory and Motor Pathways
13 The Brain, Cranial Nerves, and Sensory and Motor Pathways Lecture Presentation by Lori Garrett © 2018 Pearson Education, Inc. Note to the Instructor: For the third edition of Visual Anatomy & Physiology, we have updated our PowerPoints to fully integrate text and art. The pedagogy now more closely matches that of the textbook. The goal of this revised formatting is to help your students learn from the art more effectively. However, you will notice that the labels on the embedded PowerPoint art are not editable. You can easily import editable art by doing the following: Copying slides from one slide set into another You can easily copy the Label Edit art into the Lecture Presentations by using either the PowerPoint Slide Finder dialog box or Slide Sorter view. Using the Slide Finder dialog box allows you to explicitly retain the source formatting of the slides you insert. Using the Slide Finder dialog box in PowerPoint: 1. Open the original slide set in PowerPoint. 2. On the Slides tab in Normal view, click the slide thumbnail that you want the copied slides to follow. 3. On the toolbar at the top of the window, click the drop down arrow on the New Slide tab. Select Reuse Slides. 4. Click Browse to look for the file; in the Browse dialog box, select the file, and then click Open. 5. If you want the new slides to keep their current formatting, in the Slide Finder dialog box, select the Keep source formatting checkbox. When this checkbox is cleared, the copied slides assume the formatting of the slide they are inserted after.