Proceedings Expert Meeting on Critical 47 Limits for Heavy Metals And
Total Page:16
File Type:pdf, Size:1020Kb
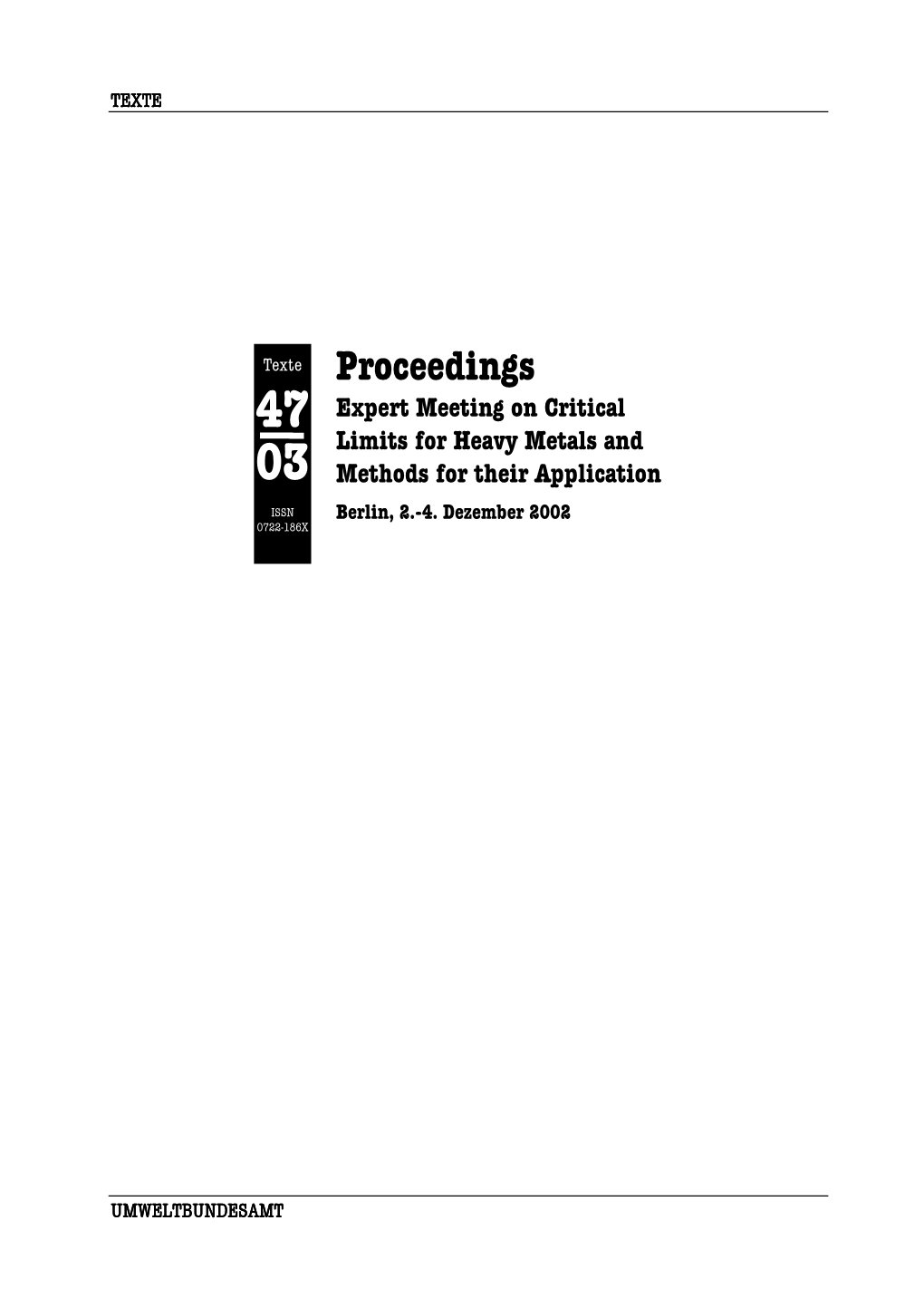
Load more
Recommended publications
-
Pollutants and Hedgehogs to Increased Numbers of Rabbits, I.E
herbivores do not have this problem as they can European rabbits have been intensively studied, move to areas where more food is available. especially with relation to social interactions and The central-place foragers’ dilemma can be re- disease ecology little was known about their for- solved when free-ranging large herbivores lower aging behaviour. This is even more the case for the vegetation height, resulting in a higher intake almost all other species of central-place forag- by rabbits, creating a facilitative effect (Chapter ing herbivores. This thesis therefore represents 5). Dekker models this relationship in space, i.e. an important contribution in understanding the he predicts that facilitation only occurs in areas ecology of this group of mammals. which sustain a high plant production. When pro- duction is too low central-place foragers do not Liesbeth Bakker benefit from the presence of free-ranging large herbivores as they are competing for the same Department of Plant-Animal Interactions limited food source. Netherlands Institute of Ecology (NIOO-CL) In the synthesis (Chapter 8), Dekker expands P.O. Box 1299 on this facilitation effect. Facilitation of small 3631 AC Maarssen herbivores by large ones has been shown to oc- The Netherlands cur in the field, but only in the sense of increased e-mail: [email protected] patch use by rabbits or other small herbivores. However, it would be very interesting to know whether this increased patch use eventually leads Pollutants and hedgehogs to increased numbers of rabbits, i.e. facilitation at the population level. Dekker’s model ad- dresses and predicts this issue, which is a highly Nondestructive exposure and risk assess relevant question for nature conservation. -
Aminopyralid Ecological Risk Assessment Final
Aminopyralid Ecological Risk Assessment Final U.S. Department of the Interior Bureau of Land Management Washington, D.C. December 2015 EXECUTIVE SUMMARY EXECUTIVE SUMMARY The United States Department of the Interior (USDOI) Bureau of Land Management (BLM) administers about 247.9 million acres in 17 western states in the continental United States (U.S.) and Alaska. One of the BLM’s highest priorities is to promote ecosystem health, and one of the greatest obstacles to achieving this goal is the rapid expansion of invasive plants (including noxious weeds and other plants not native to an area) across public lands. These invasive plants can dominate and often cause permanent damage to natural plant communities. If not eradicated or controlled, invasive plants will jeopardize the health of public lands and the activities that occur on them. Herbicides are one method employed by the BLM to control these plants. In 2007, the BLM published the Vegetation Treatments Using Herbicides on Bureau of Land Management Lands in 17 Western States Programmatic Environmental Impact Statement (17-States PEIS). The Record of Decision (ROD) for the 17-States PEIS allowed the BLM to use 18 herbicide active ingredients available for a full range of vegetation treatments in 17 western states. In the ROD, the BLM also identified a protocol for identifying, evaluating, and using new herbicide active ingredients. Under the protocol, the BLM would not be allowed to use a new herbicide active ingredient until the agency 1) assessed the hazards and risks from using the new herbicide active ingredient, and 2) prepared an Environmental Impact Statement (EIS) under the National Environmental Policy Act to assess the impacts of using new herbicide active ingredient on the natural, cultural, and social environment. -
Shrubland Ecotones Proceedings RMRS-P-11 September1999 Abstract
Some pages in this file were created by scanning the printed publication. Errors identified by the software have been corrected; however, some errors may remain. United States Department of Agriculture Proceedings: Forest Service Rocky Mountain Research Station Shrubland Ecotones Proceedings RMRS-P-11 September1999 Abstract McArthur, E. Durant; Ostler, W. Kent; Wambolt, Carl L., comps. 1999. Proceedings: shrubland ecotones; 1998 August 12–14; Ephraim, UT. Proc. RMRS-P-11. Ogden, UT: U.S. Department of Agriculture, Forest Service, Rocky Mountain Research Station. 299 p. The 51 papers in this proceedings include an introductory keynote paper on ecotones and hybrid zones and a final paper describing the mid-symposium field trip as well as collections of papers on ecotones and hybrid zones (15), population biology (6), community ecology (19), and community rehabilitation and restoration (9). All of the papers focus on wildland shrub ecosystems; 14 of the papers deal with one aspect or another of sagebrush (subgenus Tridentatae of Artemisia) ecosystems. The field trip consisted of descriptions of biology, ecology, and geology of a big sagebrush (Artemisia tridentata) hybrid zone between two subspecies (A. tridentata ssp. tridentata and A. t. ssp. vaseyana) in Salt Creek Canyon, Wasatch Mountains, Uinta National Forest, Utah, and the ecotonal or clinal vegetation gradient of the Great Basin Experimental Range, Manti-La Sal National Forest, Utah, together with its historical significance. The papers were presented at the 10th Wildland Shrub Symposium: Shrubland Ecotones, at Snow College, Ephraim, UT, August 12–14, 1998. Keywords: wildland shrubs, ecotone, hybrid zone, population biology, community ecology, restoration, rehabilitation. Acknowledgments The symposium, field trip, and subsequent publication of these proceedings were facilitated by many people and organizations. -
Draft Addendum Damage Assessment Plan for Southeast Missouri Lead Mining District: Madison County Mines Site
DRAFT ADDENDUM DAMAGE ASSESSMENT PLAN FOR SOUTHEAST MISSOURI LEAD MINING DISTRICT: MADISON COUNTY MINES SITE May 2015 Prepared for: State of Missouri Missouri Department of Natural Resources U.S. Fish and Wildlife Service U.S. Department of the Interior Prepared by: Kathy Rangen Missouri Department of Natural Resources Jefferson City, MO 65101 David E. Mosby U.S. Fish and Wildlife Service U.S. Department of the Interior Columbia, MO 65203 TABLE OF CONTENTS EXECUTIVE SUMMARY 1 CHAPTER 1 INTRODUCTION 5 1.1 Madison County Mines Site Description 5 1.1.1 Response Activities at the MCM Superfund Site 7 1.2 Natural Resource Damage Assessment Activities at MCM Site 10 CHAPTER 2 AFFECTED NATURAL RESOURCES IN THE MADISON 11 COUNTY MINES SITE 2.1 Surface Water Resources: Rivers and Streams 11 2.1.1 St. Francois River and Tributaries Surface Water 12 2.2 Geologic Resources 13 2.3 Ground Water 14 2.4 Biotic Resources 15 2.4.1 Threatened and Endangered Species 15 2.4.2 Vegetation 17 2.4.3 Aquatic and Amphibious Species 18 2.4.4 Birds 19 2.4.5 Mammals 20 2.5 Contaminants of Concern 20 2.5.1 Cadmium 21 2.5.2 Lead 21 2.5.3 Zinc 22 2.5.4 Copper 22 2.5.5 Nickel 23 2.6 Confirmation of Exposure 23 2.6.1 Surface Water 23 2.6.2 Geologic Resources 23 2.6.3 Ground Water 25 2.6.4 Biotic Resources 26 2.7 Preliminary Determination of Recovery Period 24 2.8 Quality Assurance Management 25 CHAPTER 3 OVERVIEW OF CURRENTLY PROPOSED AND/OR 25 CONTEMPLATED STUDIES REFERENCES 30 LIST OF EXHIBITS Exhibit ES-1 Currently Anticipated Madison County Mines Site NRDAR 4 Studies -
Diquat Ecological Risk Assessment, Final Report
Utah State University DigitalCommons@USU All U.S. Government Documents (Utah Regional U.S. Government Documents (Utah Regional Depository) Depository) 11-2005 Diquat Ecological Risk Assessment, Final Report Bureau of Land Management Follow this and additional works at: https://digitalcommons.usu.edu/govdocs Part of the Life Sciences Commons, and the Physical Sciences and Mathematics Commons Recommended Citation Bureau of Land Management, "Diquat Ecological Risk Assessment, Final Report" (2005). All U.S. Government Documents (Utah Regional Depository). Paper 107. https://digitalcommons.usu.edu/govdocs/107 This Report is brought to you for free and open access by the U.S. Government Documents (Utah Regional Depository) at DigitalCommons@USU. It has been accepted for inclusion in All U.S. Government Documents (Utah Regional Depository) by an authorized administrator of DigitalCommons@USU. For more information, please contact [email protected]. Bureau of Land Management Reno, Nevada Diquat Ecological Risk Assessment Final Report November 2005 Bureau of Land Management Contract No. NAD010156 ENSR Document Number 09090-020-650 Executive Summary The United States Department of the Interior (USDI) Bureau of Land Management (BLM) is proposing a program to treat vegetation on up to six million acres of public lands annually in 17 western states in the continental United States (US) and Alaska. As part of this program, the BLM is proposing the use of ten herbicide active ingredients (a.i.) to control invasive plants and noxious weeds on approximately one million of the 6 million acres proposed for treatment. The BLM and its contractor, ENSR, are preparing a Vegetation Treatments Programmatic Environmental Impact Statement (EIS) to evaluate this and other proposed vegetation treatment methods and alternatives on lands managed by the BLM in the western continental US and Alaska. -
Freeandfreak Ysince
FREEANDFREAKYSINCE | DECEMBER THIS WEEK CHICAGOREADER | DECEMBER | VOLUME NUMBER IN THIS ISSUE TR - YEARINREVIEW 20 TheInternetTheyearofTikTok theWorldoff erstidylessonson “bootgaze”crewtheKeenerFamily @ 04 TheReaderThestoryof 21 DanceInayearoflosswefound Americanpowerdynamicsand returnwithasecondEP astoldthroughsomeofourfavorite thatdanceiseverywhere WildMountainThymefeaturesone PPTB covers 22 TheaterChicagotheaterartists ofthemostagonizingcourtshipsin OPINION PECKH 06 FoodChicagorestaurantsate rosetochallengesandcreated moviehistory 40 NationalPoliticsWhen ECS K CLR H shitthisyearAlotofshitwasstill newonesin politiciansselloutwealllose GD AH prettygreat 24 MoviesRelivetheyearinfi lm MUSIC &NIGHTLIFE 42 SavageLoveDanSavage MEP M 08 Joravsky|PoliticsIthinkwe withthesedoublefeatures 34 ChicagoansofNoteDoug answersquestionsaboutmonsters TDEKR CEBW canallagreethenextyearhasgot 28 AlbumsThebestoverlooked Maloneownerandleadengineer inbedandmothersinlaw AEJL tobebetter Chicagorecordsof JamdekRecordingStudio SWMD LG 10 NewsOntheviolencesadness 30 GigPostersTheReadergot 35 RecordsofNoteApandemic DI BJ MS CLASSIFIEDS EAS N L andhopeof creativetofi ndwaystokeep can’tstopthemusicandthisweek 43 Jobs PM KW 14 Isaacs|CultureSheearned upli ingChicagoartistsin theReaderreviewscurrentreleases 43 Apartments&Spaces L CSC-J thetitlestillhewasdissingher! 32 MusiciansThemusicscene byDJEarltheMiyumiProject 43 Marketplace SJR F AM R WouldhedothesametosayDr doubleddownonmutualaidand FreddieOldSoulMarkLanegan CEBN B Kissinger? fundraisingforcommunitygroups -
Whiskey Strings Tour
K k ROCKTOBER 2017 K g VOL. 29 #9 H WOWHALL.ORGk artist, and newly graduated with (Sara Bareilles, Tori Amos) and a Bachelors of Music Composition Benny Cassette (Kanye West) in from Cornish College of the Arts, 2014. Her smash single “Secrets” she had begun to establish herself launched to No. 1 on the Billboard MARY around the Seattle area performing Dance charts, and was certified slam poetry and fusing a talk- RIAA Gold in 2015. The New singing style into her intimate York Times called her debut album performances. She received a “refreshing and severely personal.” LAMBERT phone call from a friend who was All though the success, Mary working with Macklemore and had her inner struggles. Ryan Lewis on their debut album Lambert was raised in an The Heist. Macklemore and Lewis abusive home, attempted suicide IS A were struggling to write a chorus at 17, turned to drugs and alcohol for their new song, a marriage- before being diagnosed with equality anthem called “Same bipolar disorder, and survived Love”. Lambert had three hours multiple sexual assaults throughout BABE to write the hook, and the result her childhood. With that list of was the transcendent and beautiful horrors, you wouldn’t expect Mary chorus to Macklemore & Ryan to be disarmingly joyful, but she (AND SO ARE YOU) Lewis’ triple-platinum hit “Same charms effortlessly, and the effect Love”, which Lambert wrote from on her audience is bewitching. her vantage point of being both a She describes her performances Christian and a lesbian. as, “safe spaces where crying is Writing and singing the hook encouraged.” Mary Lambert says, led to two Grammy nominations “My entire prerogative is about for “Song Of The Year” and connection, about being present, By Maya Vagner Mal Blum. -
30832 Luftkrigsskolen 29
UAV – bare ny teknologi eller en ny strategisk virkelighet? Luftkrigsskolens skriftserie Vol. 29 Andre utgivelser i skriftserien: Vol. 1 Luftforsvaret – et flerbruksverktøy for den kalde krigen? (1999) Øistein Espenes og Nils Naastad. Vol. 2 Aspekter ved konflikt og konflikthåndtering i Kosovo (2000) Gunnar Fermann Vol. 3 Nytt NATO – nytt Luftforsvar?: GILs luftmaktseminar 2000 (2000) Lars Fredrik Moe Øksendal (red.) Vol. 4 Luftkampen sett og vurdert fra Beograd (2000) Ljubisa Rajik Vol. 5 Luftforsvaret i fremtiden: nisjeverktøy for NATO eller multiverktøy for Norge? (2001) John Andreas Olsen Vol. 6 Litteratur om norsk luftfart før 2. verdenskrig: en oversikt og bibliografi (2001) Ole Jørgen Maaø Vol. 7 A critique of the Norwegian air power doctrine (2002) Albert Jensen og Terje Korsnes Vol. 8 Luftmakt, Luftforsvarets og assymetriens utfordringer. GILs luftmaktseminar 2002 (2002) Karl Erik Haug (red.) Vol. 9 Krigen mot Irak: noen perspektiver på bruken av luftmakt (2003) Morten Karlsen, Ole Jørgen Maaø og Nils Naastad Vol. 10 Luftmakt 2020: fremtidige konflikter. GILs luftmaktseminar 2003 (2003) Karl Selanger (red.) Vol. 11 Luftforsvaret og moderne transformasjon: dagens valg, morgendagens tvangstrøye? (2003) Ole Jørgen Maaø (red.) Vol. 12 Luftforsvaret i krig: ledererfaringer og menneskelige betraktninger. GILs lederskapsseminar 2003 (2003) Bjørn Magne Smedsrud (red.) Vol. 13 Strategisk overraskelse sett i lys av Weserübung, Pearl Harbor og Oktoberkrigen (2005) Steinar Larsen Vol. 14 Luftforsvaret i Kongo 1960–1964 (2005) Ståle Schirmer-Michalsen (red.) Vol. 15 Luftforsvarets helikopterengasjement i internasjonale operasjoner: et historisk tilbakeblikk (2005) Ståle Schirmer-Michalsen Vol. 16 Nytt kampfly – Hvilket og til hva? GILs luftmaktseminar 2007 (2007) Torgeir E. Sæveraas (red.) Vol. 17 Trenchard and Slessor: On the Supremacy of Air Power over Sea Power (2007) Gjert Lage Dyndal Vol. -
Affected Environment and Environmental Consequences
BLM U.S. Department of the Interior Bureau of Land Management Land Speed Record Challenger North American Eagle, Inc. Diamond Valley, Eureka County, Nevada Final Environmental Assessment DOI-BLM-NV-B010-2016-0018-EA Preparing Office Battle Mountain District Office 50 Bastian Road Battle Mountain, NV 89820 December 2017 This page intentionally left blank. TABLE OF CONTENTS i Table of Contents Chapter One: Purpose and Need for Action ............................................................... 1 1.0 Introduction ......................................................................................................... 3 Purpose and Need for Action .............................................................................. 3 1.2 Decision to be Made ........................................................................................... 6 1.3 Public Scoping Issues Identified .......................................................................... 6 1.3.1 Relevant Issues....................................................................................................... 6 1.4 BLM Responsibilities and Relationship to Planning ............................................. 7 1.4.1 Conformance to Plans, Statutes, and Regulations ................................................. 7 Chapter Two: Management Alternatives ................................................................... 11 2.0 Introduction ........................................................................................................13 2.1 Proposed Action: Land -
Patterns in Bird Community Structure Related to Restoration of Minnesota Dry Oak Savannas and Across a Prairie to Oak Woodland E
RESEARCH ARTICLE ABSTRACT: There is limited understanding of the influence of fire and vegetation structure on bird communities in dry oak (Quercus spp.) savannas of the Upper Midwest and whether bird communities in restored savanna habitats are similar to those in remnant savannas. During the 2001 and 2002 breeding seasons, we examined the relationship between bird communities and environmental variables, includ • ing vegetation characteristics and site prescribed-burn frequencies, across a habitat gradient in dry oak savannas in central Minnesota. The habitat gradient we studied went from: (1) prairie to (2) remnant Patterns in bird oak savanna to (3) oak woodland undergoing savanna restoration via fire or mechanical removal of woody vegetation to (4) oak woodland. We conducted fixed-radius point counts (n = 120) within habitats community structure with either prairie groundcover or predominately oak canopy. We described canopy and groundcover characteristics at a sub-sample (n = 28) of non-prairie points, and collected canopy and woody species related to restoration richness data and prescribed-bum frequencies over the past 20 years for all points. Observed bird com munities were most strongly correlated with canopy cover and bum frequency and, to a lesser extent, of Minnesota dry oak attributes of the shrub component. Most savanna points had bird communities that were distinct from those found at oak woodland or oak woodland points undergoing restoration via burning. Savanna points savannas and across similar to oak woodland points were in areas managed by periodic cutting rather than burning. Remnant savanna bird communities were more strongly associated with prescribed burning than those in other a prairie to oak habitat types, but it appeared that most oak woodlands that had undergone ~ 20 years of prescribed burning remained ecologically distinct from remnant savannas. -
Foraging Guilds of North American Birds
RESEARCH Foraging Guilds of North American Birds RICHARD M. DE GRAAF ABSTRACT / We propose a foraging guild classification for USDA Forest Service North American inland, coastal, and pelagic birds. This classi- Northeastern Forest Experiment Station fication uses a three-part identification for each guild--major University of Massachusetts food, feeding substrate, and foraging technique--to classify Amherst, Massachusetts 01003, USA 672 species of birds in both the breeding and nonbreeding seasons. We have attempted to group species that use similar resources in similar ways. Researchers have identified forag- NANCY G. TILGHMAN ing guilds generally by examining species distributions along USDA Forest Service one or more defined environmental axes. Such studies fre- Northeastern Forest Experiment Station quently result in species with several guild designations. While Warren, Pennsylvania 16365, USA the continuance of these studies is important, to accurately describe species' functional roles, managers need methods to STANLEY H. ANDERSON consider many species simultaneously when trying to deter- USDI Fish and Wildlife Service mine the impacts of habitat alteration. Thus, we present an Wyoming Cooperative Wildlife Research Unit avian foraging classification as a starting point for further dis- University of Wyoming cussion to aid those faced with the task of describing commu- Laramie, Wyoming 82071, USA nity effects of habitat change. Many approaches have been taken to describe bird Severinghaus's guilds were not all ba~cd on habitat feeding behavior. Comparisons between different studies, requirements, to question whether the indicator concept however, have been difficult because of differences in would be effective. terminology. We propose to establish a classification Thomas and others (1979) developed lists of species scheme for North American birds by using common by life form for each habitat and successional stage in the terminology based on major food type, substrate, and Blue Mountains of Oregon. -
Page 1 of 163 Music
Music Psychedelic Navigator 1 Acid Mother Guru Guru 1.Stonerrock Socks (10:49) 2.Bayangobi (20:24) 3.For Bunka-San (2:18) 4.Psychedelic Navigator (19:49) 5.Bo Diddley (8:41) IAO Chant from the Cosmic Inferno 2 Acid Mothers Temple 1.IAO Chant From The Cosmic Inferno (51:24) Nam Myo Ho Ren Ge Kyo 3 Acid Mothers Temple 1.Nam Myo Ho Ren Ge Kyo (1:05:15) Absolutely Freak Out (Zap Your Mind!) 4 Acid Mothers Temple & The Melting Paraiso U.F.O. 1.Star Child vs Third Bad Stone (3:49) 2.Supernal Infinite Space - Waikiki Easy Meat (19:09) 3.Grapefruit March - Virgin UFO – Let's Have A Ball - Pagan Nova (20:19) 4.Stone Stoner (16:32) 1.The Incipient Light Of The Echoes (12:15) 2.Magic Aum Rock - Mercurical Megatronic Meninx (7:39) 3.Children Of The Drab - Surfin' Paris Texas - Virgin UFO Feedback (24:35) 4.The Kiss That Took A Trip - Magic Aum Rock Again - Love Is Overborne - Fly High (19:25) Electric Heavyland 5 Acid Mothers Temple & The Melting Paraiso U.F.O. 1.Atomic Rotary Grinding God (15:43) 2.Loved And Confused (17:02) 3.Phantom Of Galactic Magnum (18:58) In C 6 Acid Mothers Temple & The Melting Paraiso U.F.O. 1.In C (20:32) 2.In E (16:31) 3.In D (19:47) Page 1 of 163 Music Last Chance Disco 7 Acoustic Ladyland 1.Iggy (1:56) 9.Thing (2:39) 2.Om Konz (5:50) 10.Of You (4:39) 3.Deckchair (4:06) 11.Nico (4:42) 4.Remember (5:45) 5.Perfect Bitch (1:58) 6.Ludwig Van Ramone (4:38) 7.High Heel Blues (2:02) 8.Trial And Error (4:47) Last 8 Agitation Free 1.Soundpool (5:54) 2.Laila II (16:58) 3.Looping IV (22:43) Malesch 9 Agitation Free 1.You Play For