Estimating the Thermodynamic Properties of Phosphate Minerals At
Total Page:16
File Type:pdf, Size:1020Kb
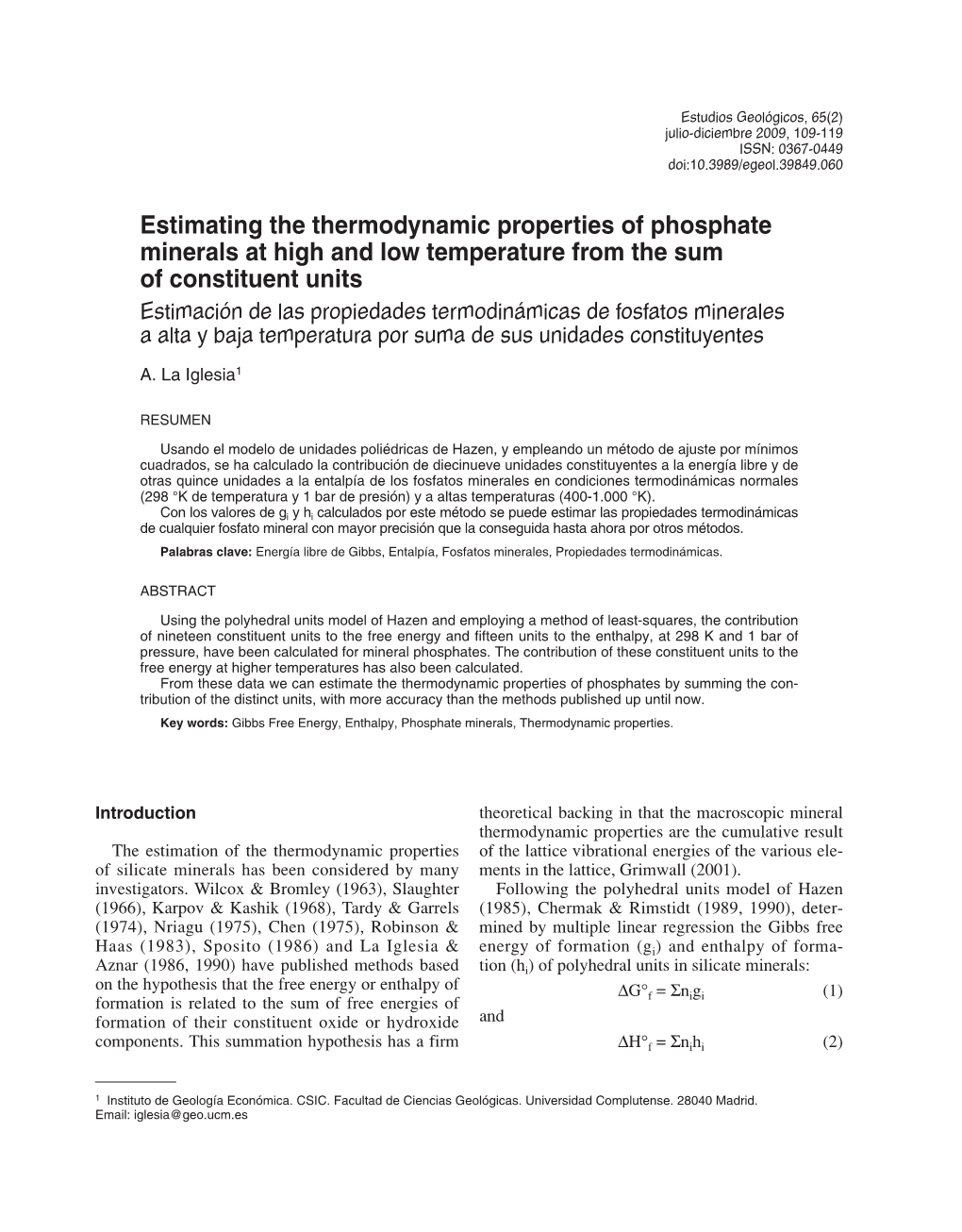
Load more
Recommended publications
-
Precipitation of Phosphate Minerals from Effluent of Anaerobically Digested Swine Manure Alex Y
University of South Florida Scholar Commons Graduate Theses and Dissertations Graduate School January 2012 Precipitation of Phosphate Minerals from Effluent of Anaerobically Digested Swine Manure Alex Y. Lin University of South Florida, [email protected] Follow this and additional works at: http://scholarcommons.usf.edu/etd Part of the Chemical Engineering Commons, Environmental Engineering Commons, and the Environmental Sciences Commons Scholar Commons Citation Lin, Alex Y., "Precipitation of Phosphate Minerals from Effluent of Anaerobically Digested Swine Manure" (2012). Graduate Theses and Dissertations. http://scholarcommons.usf.edu/etd/4359 This Thesis is brought to you for free and open access by the Graduate School at Scholar Commons. It has been accepted for inclusion in Graduate Theses and Dissertations by an authorized administrator of Scholar Commons. For more information, please contact [email protected]. Precipitation of Phosphate Minerals from Effluent of Anaerobically Digested Swine Manure by Alex Yuan-li Lin A thesis submitted in partial fulfillment of the requirements for the degree of Master of Science Department of Civil and Environmental Engineering College of Engineering University of South Florida Co-Major Professor: Sarina Ergas, Ph.D. Co-Major Professor: Jeffrey Cunningham, Ph.D. Maya Trotz, Ph.D. Date of Approval: November 9, 2012 Keywords: struvite, wastewater, confined animal feeding operation (CAFO), fertilizer, synthetic Copyright © 2012, Alex Yuan-li Lin DEDICATION I dedicate this thesis to all those that have supported me along the way whether directly or indirectly. I would like to thank members of Intervarsity on the USF campus, members of Community Life Church, and my family for their support and encouragement in many ways. -
Brushite and Taranakite from Pig Hole Cave, Giles County, Virginia
BRUSHITE AND TARANAKITE FROM PIG HOLE CAVE, GILES COUNTY, VIRGINIA Jonw W. MunnAv, Department oJ Chemistry, and Rrcnano V. Drrrnrcu, Departmenl.of Geo- logical S ciences,V ir ginia P olytechnicI nstitute, Blacksburg, Virginia. Ansrnacr Brushite occurs as nodular massesof platy crystals in the lower part of a bat guano and hair deposit in Pig Hole Cave, Giles County, Virginia. Taranakite occurs as flour-like masses near contacts between the guano and hair deposit and clay and along fractures within brecciated clay within the same cave. Chemical, physical, optical, r-ray, and thermal data for each of these minerals are presented. Some of the thermal data for brushite ano many of the data for taranakite are reported here for the first time. It is suggested that the taranakite formed as the result of reactions between the bat guano and bat hair and clay. Iwrnorucuow The presenceof the minerals later identified as brushite and taranakite in a bat guano and hair deposit in Pig Hole Cave, Giles County, Virginia, was discoveredin January, 1954. So far as has been ascertainedby a search of the literature, this is the first reported natural occurrence of either brushite or taranakite in the United States of America. After posi- tive identification of the minerals, further investigations of them were undertaken mainly becauseof their relative abundance within the cave. Locar,try AND OccURRENCES Pig Hole Cave is located beneath the property of A. B. Porterfield on Johns Creek Mountain in southeastern Giles County, southwestern Virginia. The cave was so named becauseof the former presencewithin it of the odoriferous remains of a pig lodged in a crawlway beneath the natural opening to the cave. -
Mineralogical Reconnaissance of Caves from Mallorca Island
ENDINS, núm. 27. 2005. Mallorca MINERALOGICAL RECONNAISSANCE OF CAVES FROM MALLORCA ISLAND by Bogdan P. ONAC 1, Joan J. FORNÓS 2, Àngel GINÉS 3 and Joaquín GINÉS 2 Resum S’han fet prospeccions des d’un punt de vista mineralògic a divuit cavitats de l’illa de Mallorca. Han estat identificats, mitjançant anàlisis de difracció de raigs-X, infraroigs, tèrmics i microscopia electrònica (SEM), 16 minerals que s’engloben dins de quatre grups químics diferents. La calcita ha estat l’únic mineral present a totes les cavitats prospeccionades. En espeleotemes de quatre coves diferents s’ha iden- tificat aragonita, guix i hidroxilapatita. Endemés, també han estat identificats alguns altres minerals dels grups dels carbonats, fosfats i silicats, presents en forma de crostes, cristalls diminuts o masses terroses. Els mecanismes responsables de la deposició mineral en les coves de Mallorca són: (i) precipitació a partir de l’aigua de percolació, (ii) precipitació en la zona de mescla (aigua dolça – aigua marina), (iii) reacció entre la roca encaixant i diversos espeleotemes, i les solucions enriquides en fosfats procedents del guano de les rates pinyades, i (iv) transició de fases mi- nerals. Des del punt de vista de la mineralogia, la Cova de sa Guitarreta i la Cova de ses Rates Pinyades s’han confirmat com a dues de les coves més destacables; cada una d’elles presenta vuit autèntics minerals de cova. L’associació de fosfats que contenen és diversa i interessant. Abstract Eighteen caves on the Mallorca Island were investigated with respect to their mineralogy. Sixteen minerals, divided into four chemical groups, were identified and described using X-ray diffraction, infrared, thermal, and scanning electron microsco- pe analyses. -
DESCRIPTIVE HUMAN PATHOLOGICAL MINERALOGY 1179 but Still Occursregularly
Amerkan Mincraloght, Volume 59, pages I177-1182, 1974 DescriptiveHuman Pathological Mineralogy Rrcneno I. Gmsox P.O. Box I O79, Dauis,C alilornia 95 6 I 6 Absfract Crystallographic, petrographic, and X-ray powder difiraction analysis of approximately 15,000 samples showed that the most common mineral constituents of human pathological concretions are calcium oxalates (whewellite and weddellite), calcium phosphates (apatite, brushite, and whitlockite), and magnesium phosphates (struvite and newberyite). Less are monetite, hannayite, calcite, aragonite, vaterite, halite, gypsum, and hexahydrite."o-rnon of the variables determining which minerals precipitate, the effects of different pH values on deposi- tional conditions are most apparent, and are shown by occurrences and relationships among many of the minerals studied. A pH-sensitive series has been identified among magnesium phosphatesin concretions. Introduction The study was carried out over a period of three The importanceof mineralogyin the field of medi- years.Composition was confirmedby X-ray powder cine lies in the applicationof mineralogicalmethods diffraction and polarizing microscopy;sequence was to study pathologicalmineral depositsin the human arrived at from considerationsof microscopic tex- body. Urology benefitsgreatly becauseconcretions tural and crystallographicrelationships. More than of mineral matter (calculi) are common in the 14,500samples were derivedfrom the urinary sys- urinary system.The value of mineralogicalanalysis tem of kidneys,ureters, bladder, and urethra; the of urinary material was first describedby prien and remaining samples are not statistically significant Frondel (1947). Mineralogistsmay be unawareof and arediscussed only briefly. the variability and nature of such compounds be- Calcium cause reports are usually published in medical Oxalates journals. This investigationreports the mineralogy Whewellite, CaCzOE.H2O,and weddellite, CaCz- and possiblepathological significanceof these min- O4'2H2O,are very uncommonin the mineralworld. -
1 Raman Spectroscopy of Newberyite, Hannayite and Struvite. Ray L. Frost
Raman spectroscopy of newberyite, hannayite and struvite. Ray L. Frost• a, Matt L. Weier a, Wayde N. Martens, a Dermot A. Henry b, and Stuart J. Mills b,c a Inorganic Materials Research Program, School of Physical and Chemical Sciences, Queensland University of Technology, GPO Box 2434, Brisbane Queensland 4001, Australia. b Geosciences, Museum Victoria, PO Box 666E, Melbourne, Victoria 3001, Australia. c CSIRO Minerals, Box 312, Clayton South, Victoria 3169, Australia. This is the authors’ version of a paper that was later published as: Frost, Ray, Weier, Matt , Martens, Wayde, Henry, Dermot & Mills, Stuart (2005) Raman spectroscopy of newberyite, hannayite and struvite. Spectrochimica Acta 62(1):pp. 181-188. Copyright 2005 Elsevier Abstract The phosphate minerals hannayite, newberyite and struvite have been studied by Raman spectroscopy using a thermal stage. Hannayite and newberyite are -1 characterised by an intense band at around 980 cm assigned to the ν1 symmetric stretching vibration of the HPO4 units. In contrast the symmetric stretching mode is -1 observed at 942 cm for struvite. The Raman spectra are characterised by multiple ν3 antisymmetric stretching bands and ν2 and ν4 bending modes indicating strong distortion of the HPO4 and PO4 units. Hannayite and newberyite are defined by bands -1 at 3382 and 3350 cm attributed to HOPO3 vibrations and hannayite and struvite by + bands at 2990, 2973 and 2874 assigned to NH4 bands. Raman spectroscopy has proven most useful for the analysis of these ‘cave’ minerals where complex paragenetic relationships exist between the minerals. Keywords: hannayite, newberryite, struvite, phosphate, Raman spectroscopy Introduction Interest in struvite formation also comes from the formation in urinary tracts and kidneys [1-6]. -
Bladder Stones in Dogs & Cats By: Dr
Navarro Small Animal Clinic 5009 Country Club Dr. Victoria, TX 77904 361-573-2491 www.navarrosmallanimalclinic.com Bladder Stones in Dogs & Cats By: Dr. Shana Bohac Dogs, like people, can develop a variety of bladder stones. These stones are rock-like structures that are formed by minerals. Some stones form in alkaline urine, whereas others form when the urine is more acidic. Bladder stones are very common in dogs, particularly small breed dogs. The most common signs that a dog or cat has bladder stones include blood in the urine, and straining to urinate. Blood is seen due to the stones bouncing around and hitting the bladder wall. This can irritate and damage the tissue and can cause cystitis (inflammation of the bladder). Straining to urinate occurs because of the inflammation and irritation of the bladder walls or urethra or muscle spasms. The stone itself can actually obstruct the flow of urine if it blocks the urethra. Small stones can get stuck in the urethra and cause a complete obstruction. This can be life threatening if the obstruction is not relieved since the bladder can rupture as more urine is produced with nowhere to go. Bladder stones form because of changes in the urine pH. Normal dog urine is slightly acidic and contains waste products such as dissolved minerals and enzymes such as urease. Urease breaks down excess ammonia in urine. An overload of ammonia in urine can cause bladder inflammation and thickening known as cystitis. There are a variety of stones that can form in the bladder, some that form in acidic urine, while others form in alkaline urine. -
Current Insights Into the Mechanisms and Management of Infection Stones
Current insights into the mechanisms and management of infection stones Authors: Erika J. Espinosa-Ortiz, Brian H. Eisner, Dirk Lange, and Robin Gerlach The final publication is available at Springer via https://dx.doi.org/10.1038/s41585-018-0120-z. Espinosa-Ortiz, Erika J., Brian H. Eisner, Dirk Lange, and Robin Gerlach, “Current insights into the mechanisms and management of infection stones,” Nature Reviews Urology, November 2018, 16: 35-53. doi: 10.1038/s41585-018-0120-z. Made available through Montana State University’s ScholarWorks scholarworks.montana.edu Current insights into the mechanisms and management of infection stones Erika J. Espinosa-Ortiz1,2, Brian H. Eisner3, Dirk Lange4* and Robin Gerlach 1,2* Abstract | Infection stones are complex aggregates of crystals amalgamated in an organic matrix that are strictly associated with urinary tract infections. The management of patients who form infection stones is challenging owing to the complexity of the calculi and high recurrence rates. The formation of infection stones is a multifactorial process that can be driven by urine chemistry , the urine microenvironment, the presence of modulator substances in urine, associations with bacteria, and the development of biofilms. Despite decades of investigation, the mechanisms of infection stone formation are still poorly understood. A mechanistic understanding of the formation and growth of infection stones — including the role of organics in the stone matrix, microorganisms, and biofilms in stone formation and their effect on stone characteristics — and the medical implications of these insights might be crucial for the development of improved treatments. Tools and approaches used in various disciplines (for example, engineering, chemistry , mineralogy , and microbiology) can be applied to further understand the microorganism–mineral interactions that lead to infection stone formation. -
Struvite Urolithiasis in Dogs
Glendale Animal Hospital 623-934-7243 www.familyvet.com Struvite Urolithiasis in Dogs (Struvite Stones in the Urinary Tract of Dogs) Basics OVERVIEW • ”Urolithiasis” is the medical term for the presence of stones (known as “uroliths”) in the urinary tract • The most common minerals found in the stones (uroliths) are used to name the particular stone; in this type of stone, struvite makes up the composition of the stone, and thus the name “struvite urolithiasis”; struvite is magnesium ammonium phosphate • The urinary tract consists of the kidneys, the ureters (the tubes running from the kidneys to the bladder), the urinary bladder (that collects urine and stores it until the pet urinates), and the urethra (the tube from the bladder to the outside, through which urine flows out of the body) • Struvite urolithiasis is the formation of crystalline stones (uroliths) composed of magnesium ammonium phosphate, or struvite, in the urinary tract GENETICS • The high incidence of struvite stones (uroliths) in some breeds of dogs (such as the miniature schnauzer) suggests a familial (runs in certain families or lines of animals) tendency; it is hypothesized that susceptible miniature schnauzers inherit some abnormality of local host defenses of the urinary tract that increases their likelihood to develop urinary tract infection (UTI) • Sterile struvite uroliths were found in a family of English cocker spaniels SIGNALMENT/DESCRIPTION OF PET Species • Dogs Breed Predilections • Miniature schnauzer, shih tzu, bichon frise, miniature poodle, cocker spaniel, -
Mineralogical Data on Bat Guano Deposits from Three Romanian Caves
Studia UBB Geologia, 2013, 58 (2), 13 – 18 Mineralogical data on bat guano deposits from three Romanian caves Alexandra GIURGIU1* & Tudor TĂMAŞ1,2 1Department of Geology, Babeș-Bolyai University, Kogălniceanu 1, 400084 Cluj-Napoca, Romania 2“Emil Racoviţă” Institute of Speleology, Clinicilor 5, 400006 Cluj-Napoca, Romania Received August 2013; accepted September 2013 Available online 14 October 2013 DOI: http://dx.doi.org/10.5038/1937-8602.58.2.2 Abstract. Mineralogical studies performed on crusts, nodules and earthy masses from the Romanian caves Gaura cu Muscă, Gaura Haiducească and Peștera Zidită have revealed the presence of three different phosphate associations. The minerals have been identified by means of X-ray diffraction, scanning electron microscopy, and energy dispersive spectroscopy. Five phosphates have been identified in the samples, with hydroxylapatite the only common mineral in all the three caves. Brushite, taranakite, leucophosphite and variscite are the other phosphates identified. Associated minerals include gypsum, calcite, quartz, and illite-group minerals. Aside from differences in the lithology, the occurrences of the different phosphate minerals indicate variable pH and relative humidity conditions near or within the guano accumulations. Keywords: guano, cave phosphate associations, Gaura cu Muscă, Gaura Haiducească, Zidită Cave, Romania INTRODUCTION environmental conditions in which they have formed. Of these three caves, only Gaura cu Muscă was previously studied with respect to Phosphate minerals are a common feature in caves containing its mineralogy, and a phosphate association consisting of vashegyite, bat guano accumulations, where they form as a result of the crandallite, and ardealite was described (Onac et al., 2006). interaction of guano derived solutions with the cave bedrock or with secondary (chemical or detrital) cave deposits. -
Optical Properties of Common Rock-Forming Minerals
AppendixA __________ Optical Properties of Common Rock-Forming Minerals 325 Optical Properties of Common Rock-Forming Minerals J. B. Lyons, S. A. Morse, and R. E. Stoiber Distinguishing Characteristics Chemical XI. System and Indices Birefringence "Characteristically parallel, but Mineral Composition Best Cleavage Sign,2V and Relief and Color see Fig. 13-3. A. High Positive Relief Zircon ZrSiO. Tet. (+) 111=1.940 High biref. Small euhedral grains show (.055) parallel" extinction; may cause pleochroic haloes if enclosed in other minerals Sphene CaTiSiOs Mon. (110) (+) 30-50 13=1.895 High biref. Wedge-shaped grains; may (Titanite) to 1.935 (0.108-.135) show (110) cleavage or (100) Often or (221) parting; ZI\c=51 0; brownish in very high relief; r>v extreme. color CtJI\) 0) Gamet AsB2(SiO.la where Iso. High Grandite often Very pale pink commonest A = R2+ and B = RS + 1.7-1.9 weakly color; inclusions common. birefracting. Indices vary widely with composition. Crystals often euhedraL Uvarovite green, very rare. Staurolite H2FeAI.Si2O'2 Orth. (010) (+) 2V = 87 13=1.750 Low biref. Pleochroic colorless to golden (approximately) (.012) yellow; one good cleavage; twins cruciform or oblique; metamorphic. Olivine Series Mg2SiO. Orth. (+) 2V=85 13=1.651 High biref. Colorless (Fo) to yellow or pale to to (.035) brown (Fa); high relief. Fe2SiO. Orth. (-) 2V=47 13=1.865 High biref. Shagreen (mottled) surface; (.051) often cracked and altered to %II - serpentine. Poor (010) and (100) cleavages. Extinction par- ~ ~ alleL" l~4~ Tourmaline Na(Mg,Fe,Mn,Li,Alk Hex. (-) 111=1.636 Mod. biref. -
Tavistockite and Bialite Discredited
MINERALOGICAL MAGAZINE, MARCH 1969, VOL. 37, NO. 285 Tavistockite and bialite discredited P. G. EMBREY AND E. E. FEJER Department of Mineralogy, British Museum (Natural History) SUMMARY. Specimens of tavistockite fall into two groups: true tavistockite from the George and Charlotte mine, Tavistock, Devon, and wavellite from the Stenna Gwyn mine, St. Austell, Cornwall. Both were sold as tavistockite by the discoverer, Richard TaIling. Tavistockite proper is a fluorapatite, as shown by optical and X-ray examination, and the alumina and water in the original analysis are certainly derived from kaolinite with which the apatite is intimately associated. The published optical properties attributed to tavistockite were determined by E. S. Larsen on Stenna Gwyn material, and are those of wavellite. Re-examination of a portion of Buttgenbach's type bialite, which he related to tavistockite on optical grounds, shows it to be wavellite. TAVISTOCKITE has been a doubtful species from the time it was first described in 1865 by A. H. Church! as 'Hydrated Calcium-aluminic Phosphate (?)'. Its apparent validity has been established by successive appearances in all the standard works on systematic mineralogy, starting with J. D. Dana's renaming as tavistockite in 1868.2 The present study is perhaps as much historical as mineralogical, since Church's original material cannot be traced and other specimens present a confused picture both in naming and in locality. We have studied seventeen specimens (see table) that are or have at one time been labelled tavistockite, and find that they fall into two distinct groups that may readily be characterized by the mineral assemblages present. -
Taranakite.Pdf
3+ Taranakite (K, Na)3(Al, Fe )5(PO4)2(PO3OH)6 • 18H2O c 2001-2005 Mineral Data Publishing, version 1 Crystal Data: Hexagonal. Point Group: 32/m. Pseudohexagonal platy crystals, rarely to 0.5 mm; typically in compact nodular aggregates, pulverulent, powdery, flourlike to claylike, massive. Physical Properties: Tenacity: Unctuous. Hardness = Very soft. D(meas.) = 2.12–2.15 D(calc.) = 2.12 Optical Properties: Transparent. Color: White, gray, yellowish white; colorless in transmitted light. Optical Class: Uniaxial (–). ω = 1.506–1.510 = 1.500–1.503 Cell Data: Space Group: R3c (synthetic). a = 8.7025(11) c = 95.05(1) Z = 6 X-ray Powder Pattern: Apulian Cave PU 38, Italy. 15.82 (100), 3.82 (40), 3.14 (31), 3.36 (29), 7.47 (28), 3.59 (22), 7.92 (18) Chemistry: (1) (2) (3) (1) (2) (3) P2O5 42.32 41.88 42.30 K2O 7.09 8.43 10.53 Al2O3 19.03 17.48 18.99 H2O 31.10 28.43 28.18 Fe2O3 0.42 1.67 insol. 0.37 Na2O 1.28 Total 99.96 99.54 100.00 (1) Onino-Iwaya Cave, Japan; by gravimetry and flame photometry; corresponds to H7.09K1.97 • • (Al, Fe)4.98(PO4)8 19.7H2O. (2) Castellana Cave, Italy. (3) K3Al5(PO4)2(PO3OH)6 18H2O. Occurrence: Formed from phosphatic solutions derived from bird or bat guano reacting with clays or aluminous rocks under perenially damp conditions; the most common phosphate mineral in caves. Association: Vashegyite, leucophosphite, minyulite, francoanellite, brushite, ardealite, strengite, variscite, vivianite. Distribution: From the Sugarloaves, near New Plymouth, Taranaki Peninsula, New Zealand.