Feldspar Replacement Reactions by Interface-Coupled Dissolution-Precipitation: a Case Study from the Larvik Plutonic Complex, SE-Norway
Total Page:16
File Type:pdf, Size:1020Kb
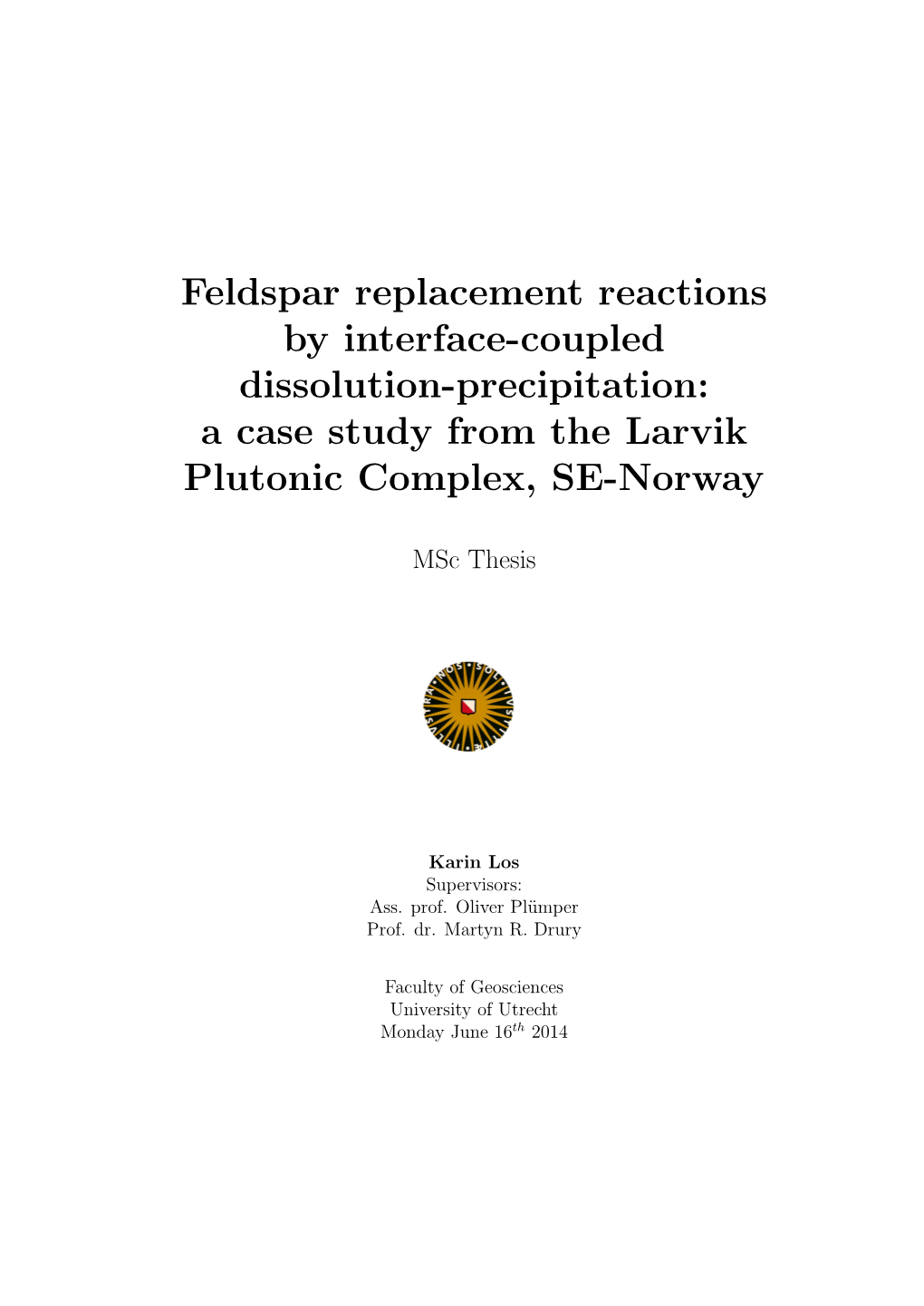
Load more
Recommended publications
-
National Treasure of Global Significance. Dimension-Stone Deposits in Larvikite, Oslo Igneous Province, Norway
National treasure of global significance. Dimension-stone deposits in larvikite, Oslo igneous province, Norway Tom Heldal1, Idunn Kjølle2, Gurli B. Meyer1 and Sven Dahlgren3 1Geological Survey of Norway (NGU), 7491 Trondheim, Norway. 2Directorate of mining, 7491 Trondheim, Norway. 3Geological advisor, Buskerud, Telemark and Vestfold, Fylkeshuset, 3126 Tønsberg, Norway. E-mail: [email protected] Larvikite has for more than a hundred years been appreciated as one of the world’s most attractive dimension stones, and at present, its production and use is more extensive than ever. The main reason for the continuous success of larvikite on the world market is the blue iridescence displayed on polished surfaces, which is caused by optical interference in microscopic lamellae within the ternary feldspars. The larvikite complex consists of different intrusions, defining several ring- shaped structures, emplaced during a period of approximately five million years. Following this pattern, several commercial subtypes of larvikite, characterised by their colour and iridescence, have been mapped. Four of these subtypes are being exploited at the present time and define the most important reserves in the short run. Some other subtypes are less attractive in the present market situation, but may provide an interesting potential for the future. However, the industrial value of the larvikite also depends on other geological features, such as various types of dykes, faults and fractures, ductile deformation zones, late-stage magmatic and hydrothermal alteration and deep weathering. When combining the distribution pattern of such features with the map of the larvikite subtypes, it is possible to delineate various types of larvikite deposit that are considered to have commercial value in the short or long term. -
The Permo-Carboniferous Oslo Rift Through Six Stages and 65 Million Years
52 by Bjørn T. Larsen1, Snorre Olaussen2, Bjørn Sundvoll3, and Michel Heeremans4 The Permo-Carboniferous Oslo Rift through six stages and 65 million years 1 Det Norske Oljeselskp ASA, Norway. E-mail: [email protected] 2 Eni Norge AS. E-mail: [email protected] 3 NHM, UiO. E-mail: [email protected] 4 Inst. for Geofag, UiO. E-mail: [email protected] The Oslo Rift is the northernmost part of the Rotliegen- des basin system in Europe. The rift was formed by lithospheric stretching north of the Tornquist fault sys- tem and is related tectonically and in time to the last phase of the Variscan orogeny. The main graben form- ing period in the Oslo Region began in Late Carbonif- erous, culminating some 20–30 Ma later with extensive volcanism and rifting, and later with uplift and emplacement of major batholiths. It ended with a final termination of intrusions in the Early Triassic, some 65 Ma after the tectonic and magmatic onset. We divide the geological development of the rift into six stages. Sediments, even with marine incursions occur exclusively during the forerunner to rifting. The mag- matic products in the Oslo Rift vary in composition and are unevenly distributed through the six stages along the length of the structure. Introduction The Oslo Palaeorift (Figure 1) contributed to the onset of a pro- longed period of extensional faulting and volcanism in NW Europe, which lasted throughout the Late Palaeozoic and the Mesozoic eras. Widespread rifting and magmatism developed north of the foreland of the Variscan Orogen during the latest Carboniferous and contin- ued in some of the areas, like the Oslo Rift, all through the Permian period. -
Graveyard Geology
GRAVEYARD GEOLOGY A Guide to Rocks in Graveyards and Cemeteries Wendy Kirk Department of Earth Sciences, David Cook University College London & Aldersbrook Geological Society London Geodiversity Partnership Introduction Walk around graveyards and cemeteries (in this case, those of London and the southeast of England) and it becomes apparent that, prior to the latter part of the twentieth century, many memorials were made out of just a few different rock types. These were chosen for reasons of appearance, cost, workability and ease of transport to the cemetery, as well as for resistance to weathering and dependence on local regulations. In the last few decades, a range of different, interesting and beautiful stones have appeared, many brought in from abroad, enhancing the diversity of materials used. The intention of this guide is to help a non-specialist identify the main rock types, to recognize some of the varieties and to know where some of these might have come from. Graveyards are a wonderful resource for those with an interest in geology at any level, wildlife, plants, history or sculpture. We hope you gain as much pleasure as we have done. First things first A useful place to start is to be able to distinguish between igneous, sedimentary and metamorphic rocks. Igneous rocks form from melted rock called magma. If this erupts at the surface, it is called lava. It cools and crystallizes quickly, so the grains are too small to see even with a hand lens (magnifying glass). If the lava erupt explosively to form a spray, the cooled fragments are known as volcanic ash. -
Petrology of Nepheline Syenite Pegmatites in the Oslo Rift, Norway: Zr and Ti Mineral Assemblages in Miaskitic and Agpaitic Pegmatites in the Larvik Plutonic Complex
MINERALOGIA, 44, No 3-4: 61-98, (2013) DOI: 10.2478/mipo-2013-0007 www.Mineralogia.pl MINERALOGICAL SOCIETY OF POLAND POLSKIE TOWARZYSTWO MINERALOGICZNE __________________________________________________________________________________________________________________________ Original paper Petrology of nepheline syenite pegmatites in the Oslo Rift, Norway: Zr and Ti mineral assemblages in miaskitic and agpaitic pegmatites in the Larvik Plutonic Complex Tom ANDERSEN1*, Muriel ERAMBERT1, Alf Olav LARSEN2, Rune S. SELBEKK3 1 Department of Geosciences, University of Oslo, PO Box 1047 Blindern, N-0316 Oslo Norway; e-mail: [email protected] 2 Statoil ASA, Hydroveien 67, N-3908 Porsgrunn, Norway 3 Natural History Museum, University of Oslo, Sars gate 1, N-0562 Oslo, Norway * Corresponding author Received: December, 2010 Received in revised form: May 15, 2012 Accepted: June 1, 2012 Available online: November 5, 2012 Abstract. Agpaitic nepheline syenites have complex, Na-Ca-Zr-Ti minerals as the main hosts for zirconium and titanium, rather than zircon and titanite, which are characteristic for miaskitic rocks. The transition from a miaskitic to an agpaitic crystallization regime in silica-undersaturated magma has traditionally been related to increasing peralkalinity of the magma, but halogen and water contents are also important parameters. The Larvik Plutonic Complex (LPC) in the Permian Oslo Rift, Norway consists of intrusions of hypersolvus monzonite (larvikite), nepheline monzonite (lardalite) and nepheline syenite. Pegmatites ranging in composition from miaskitic syenite with or without nepheline to mildly agpaitic nepheline syenite are the latest products of magmatic differentiation in the complex. The pegmatites can be grouped in (at least) four distinct suites from their magmatic Ti and Zr silicate mineral assemblages. -
Crystallization and Metasomatism of Nepheline Syenite Xenoliths in Quartz-Bearing Intrusive Rocks in the Permian Oslo Rift, SE Norway
Crystallization and metasomatism of nepheline syenite xenoliths in quartz-bearing intrusive rocks in the Permian Oslo rift, SE Norway TOM ANDERSEN & HENNING SØRENSEN Andersen, T. & Sørensen, H.: Crystallization and metasomatism of nepheline syenite xenoliths in quartz-bearing intrusive rocks in the Permian Oslo rift, SE Norway. Norsk Geologisk Tidsskrift, Vol. 73, pp. 250-266. Oslo 1993. ISSN 0029-196X. Small bodies of metasomatized nepheline syenite occur as xenoliths in syenitic and granitic intrusions in the Mykle area, ca. 30 km N of the Larvik pluton in the Vestfold Graben of the late Paleozoic Qslo rift of SE Norway. The nepheline syenite has a metaluminous major element composition, and its primary igneous mineralogy is: alkali feldspar + nepheline + clinopyroxene + titanite + magnetite + apatite ± amphibole. The mafic silicate minerals have lower (Na + K)/AI than comparable minerals in other fe lsic intrusions in the Oslo Rift. Gamet (grossular-andradite), analcime, sodalite, thomsonite and gonnardite occur as interstitial minerals in the )east altered parts of the nepheline syenite. The xenoliths were metasomatized as a result of interaction between nepheline syenite and younger silica-saturated to oversaturated magrnas and their associated fluids. Early, pervasive metasomatism led to breakdown of nepheline, replacement of pyroxene by biotite ± garnet and crystallization of quartz. Recrystallization took place at solidus-near temperatures (700-725°C), and was controlled by an increase in silica activity and oxygen fugacity. Titanite + magnetite were replaced by rutile + quartz + hematite + calcite at a late stage of the metasomatic history, at oxygen fugacities above the HM buffer, and T < 450°C. The xenoliths indicate the former presence of larger bodies of nepheline syenite in an area where no such rocks were known previously. -
Colour Plates
Plate 1. Top row left to right: Pink tourmaline, round brilliant cut: yellow topaz, step cut rectangle; pink tourmaline, scissor cut rectangle; Tropical Green from India- classified as a grey granite, course grained with essential minerals blue quartz and grey feldspar, accessory minerals are hornblende and mica. Middle row, left to right: Tarn from France - Quartz/biotite granite; Sapphire Brown from India - pink porphyritic granite, essential minerals quartz and orthoclase feldspar with perfect cleavage; Bahama Blue from India - course grained granite, equigran- ular, quartz and feldspar essential and hornblende as accessory; Balmoral Red from Sweden - quartz/biotite gran- ite. Bottom row, left to right: Ruby Red from India - pink pegmatite, quartz and pink felspar are essentials, no accessories; Paradiso from India - fine grained grey granite, essential minerals are quartz and orthoclase feldspar, accessories are rare with a few minute flakes of mica; Star Galaxy from India - medium grained diorite, plagioclase and pyroxene in equal pro- portions; Blue Pearl from Norway - larvikite with crystals of labradorite. Pictures of cut stones www.customgemstones.com Pictures of Rock samples Lidster stonemasons: www.lidster.co.uk Plate 2. Overall winner and feature category. Sea Stack, Cathedral Cove, New Zealand. Ignimbrite of the Whitianga Group Rhyolites. Linda McArdell Plate 3. Winner of geologically inspired landscape category: Lower lias limestone cliffs, overlying a Lower Lias platform, Southerndown, Glamorgan Lisa Duff Plate 4. Winner of Geological humour category. Bareback riding on a Carnosaurus, Scilly Isles. Pauline Kirtley Plate 5. Above: Satellite image of Tenerife showing many geological features. Much of the pink colour is man- made constructions. -
Contributions to the Mineralogy of Norway, No. 43 a Note on a New
Contributions to the mineralogy of Norway, No. 43 A Note on a New Occurrence of Baddeleyite in Larvikite from Larvik, Norway LENNART WIDENFALK & ROLAND GORBATSCHEV Widenfalk, L. & Gorbatschev, R.: A note on a new occurrence of baddeleyite in larvikite from Larvik, Norway. Contribution to the mineralogy of Norway, No. 43. Norsk Geologisk Tidsskrift, Vol. 51, pp. 193-194. Oslo 1971. L. Widenfalk & R. Gorbatschev, Department of Mineralogy and Geology, Uppsala University, Box 555, S-751 22, Uppsala l, Sweden. Baddeleyite has been discovered in larvikite from the central parts of the town of Larvik, Norway. The mineral was identified by microprobe and optical means. Microprobe analysis shows virtually pure Zr0.2, the contents of U and Th are below the limit of detectability. The optic axial angle is 2 V ( - ) = 30°, refraction is high, birefringence weak, color in thin-section is dirty yellowish-brown. Sets of twinning lamellae possibly coinciding with poorly developed cleavage run parallel to (110). The crystals are tabular Fig. l. Baddeleyite crystal in larvikite. The crystal is tabular parallel to (100). Twinning, which is distinguishable as sets of light lamellae runs parallel to (110) which is also the orientation of the terminating crystal surfaces. Crossed nicols, 135 X. 194 L. WIDENF ALK & R. GORBATSCHEV parallel to (100), other identified crystal surfaces being (110). The largest discovered crystal had a thin-section maximum diameter of 0.7 mm (Fig. 1). The mineral association of the investigated larvikite comprises alkali feldspar, oligoclase, nepheline, olivine, biotite, augite, Ca-amphibole, apatite, magnetite, and ilmenite. As is evident from this composition, the occurrence of baddeleyite in larvikite is due to the undersaturation of the rock in Si. -
Stone List and Descriptions
www.ablecrystals.com WE NORMALLY HAVE SAMPLES OF ALL OF THE FOLLOWING IN STOCK. They may be specimen, rough, tumbled, shaped or in jewelry. We will add items as we find more and ask you to contact us if you are looking for something not listed. We can often source it for you. As you can see we've added MANY NEW STONES and have not had time to post the properties but we wanted you to be aware WE ARE STOCKING THEM. Forgive us please if we are temporarily sold out of the item you want. The only items we will have pictures and prices for will be found under the Featured Products section of our website: www.ablecrystals.com. These will be special items of higher value so if you have a list of smaller items you want please email it and we will quote. Disclaimer : The metaphysical and healing properties of gems and crystals outlined in this section are for inspiration and reference only. These properties are suggested by myriad sources and are their attitudes and beliefs. We do not necessarily agree with all the attributes suggested but offer this information as "that of common belief'. In no way is this offering meant to replace diagnosis or treatment by a qualified therapist or physician. Content Source Disclaimer: The material in the crystal descriptions is a compilation of pieces often verbatim and mostly without reference as the sources include many books and many sites which very often had identical information. The material is automatically out of date anyway as the crystal consciousness is evolving as are we. -
Killala L. Alkalic Rock Complex
THESE TERMS GOVERN YOUR USE OF THIS DOCUMENT Your use of this Ontario Geological Survey document (the “Content”) is governed by the terms set out on this page (“Terms of Use”). By downloading this Content, you (the “User”) have accepted, and have agreed to be bound by, the Terms of Use. Content: This Content is offered by the Province of Ontario’s Ministry of Northern Development and Mines (MNDM) as a public service, on an “as-is” basis. Recommendations and statements of opinion expressed in the Content are those of the author or authors and are not to be construed as statement of government policy. You are solely responsible for your use of the Content. You should not rely on the Content for legal advice nor as authoritative in your particular circumstances. Users should verify the accuracy and applicability of any Content before acting on it. MNDM does not guarantee, or make any warranty express or implied, that the Content is current, accurate, complete or reliable. MNDM is not responsible for any damage however caused, which results, directly or indirectly, from your use of the Content. MNDM assumes no legal liability or responsibility for the Content whatsoever. Links to Other Web Sites: This Content may contain links, to Web sites that are not operated by MNDM. Linked Web sites may not be available in French. MNDM neither endorses nor assumes any responsibility for the safety, accuracy or availability of linked Web sites or the information contained on them. The linked Web sites, their operation and content are the responsibility of the person or entity for which they were created or maintained (the “Owner”). -
A Walking Tour of Building Stones from Senate House to Tottenham Court Road
A Walking Tour of Building Stones from Senate House to Tottenham Court Road London Illustrated News Dr Ruth Siddall UCL Earth Sciences Gower Street London WC1E 6BT r.siddall@ ucl.ac.uk Ruth Siddall, 1 UCL Earth Sciences 2012 A Walking Tour of Building Stones from Senate House to Tottenham Court Road Ruth Siddall This guide is an update of parts of Eric Robinson’s tour, originally published in 1985. Tottenham Court Road has transformed since then, but many of the stones still remain. The Walk Start Point: Senate House, University of London. 1. Senate House Senate House and Library where designed by Charles Holden and built during 1932-37. This is actually a paired-down version of Holden’s original plans which included buildings occupying the land now taken by Birkbeck College, ULU and the piazza behind these buildings, leading from Byng Place. Nevertheless Senate House is a striking building. The major building material is dressed Portland Stone which has retained a good white colour. The lower courses are of axe-dressed Cornish Granite, probably from Carnsew Quarry. The spacious foyer is both paved and clad with Tivoli Travertine. Leave Senate House via the main entrance onto Malet Street, cross over and continue straight ahead along Keppel Street, pausing at the entrance to the London School of Hygiene and Tropical Medicine on the corner of Keppel Street and Gower Street. 2. London School of Hygiene and Tropical Medicine Also built primarily of beautifully dressed Portland Stone, the London School of Hygiene and Tropical Medicine’s building is of interest architecturally. -
Urban Geology on Victoria Street, SW1
Urban Geology in London No. 10 Urban Geology on Victoria Street, SW1 Ruth Siddall & Di Clements 2013 This walk starts at Westminster Abbey and follows the north side of Victoria Street, SW1, as far as Westminster Cathedral and then returns along the south side of Victoria Street to Westminster Abbey. This walk may be supplemented by a visit to the astonishing collection of decorative stones used in Westminster Cathedral which are catalogued and described in Patrick Rogers’s excellent book (Rogers 2008). The first stop is a worthwhile diversion from Victoria Street along Tothill Street. From the Sanctuary cross over Victoria Street and pass Barclay’s Bank and turn left into Tothill Street. Cross over and walk up to Caxton House, the Department of Work & Pensions, on the corner of Matthew Parker Street. Caxton House Situated on the north side of Tothill Street, Caxton House’s distinctive stonework is visible from a distance, It was designed by architects Chapman Taylor Partnership, 1979 and won a Stone Award in 1981. The foundations of the building are Cornish granite, probably from Bodmin Moor. Otherwise, the building is clad with Portland Roach which is widely used in London, but this is a special facies from that formation. The Roach is a variety of Portland Stone wherein all the September 2013 1 Urban Geology in London No. 10 (aragonitic) shell fossils have been leached away leaving casts and moulds of the shells, typically a spiral gastropod known as the Portland Screw and the large shells of Trigonia species bivalves, known to the quarrymen as horses’ heads (or osses’ ‘eads). -
Lamellar and Patchy Intergrowths in Feldspars: Experimental
American Mineralogist, Volume 71, pages 343-355, 1986 Lamellar and patchy intergrowths in feldspars:Experimental crystallization of eutectic silicates JoN S. PrtnnsnN,I Glnv E. LorcnnN NASA JohnsonSpace Center SN4, Houston, Texas 77058 Ansrucr Coupled and noncoupled eutecticfeldspar intergrowths have been producedby dynamic crystallization experimentsin the ternary feldspar-water system.Fractionation during pla- gioclasecrystallization in nearly all compositions examined resultsin eutecticgrowth when a solute-enrichedboundary layer becomessupersaturated with K-feldspar. The growth rate of the eutectic product generally exceedsthat of monophaseefowth by a factor of l0 or higher and is an effective means of reducing thermal supercooling.Coupled intergrowths have lamellar structures that are normal to a planar, nonfaceted, solidJiquid interface. They are developedpredominantly in the {010} crystallographicplane of the host feldspar. The composite crystalline product is usually of-eutectic in composition. Variations in composition occur systematically along the growth direction and across single facets in responseto local changesin supercooling.Different crystallographicsectors show systematic compositional differencesdue to variable nucleation and growth kinetics, which often lead to hourglasspatterns superimposedon the intrgrowth structure.Uncoupled eutecticgrowth occursin the more K-rich melts. It is a result of the preferential groWh of sanidine in the crystallographica and c directions and simultaneous,coupled-lamellar oligoclase-sanidine intergrowth in the crystallographicb direction. This processleads to a patchy intergrowth with coarse,irregular phasedistributions and facetedinterfaces. The melt-grown composites have textures strikingly similar to feldspar intergrowths found in cumulus feldsparsof the Larvikite monzonite suite in the Oslo igneous province, and this similarity suggeststhat eutectic growth from near-cotectic melts may account for certain coarse feldspar inter- growths.