A NEW THEORY of PROTOPLASMIC STREAMING by Odis Hayden
Total Page:16
File Type:pdf, Size:1020Kb
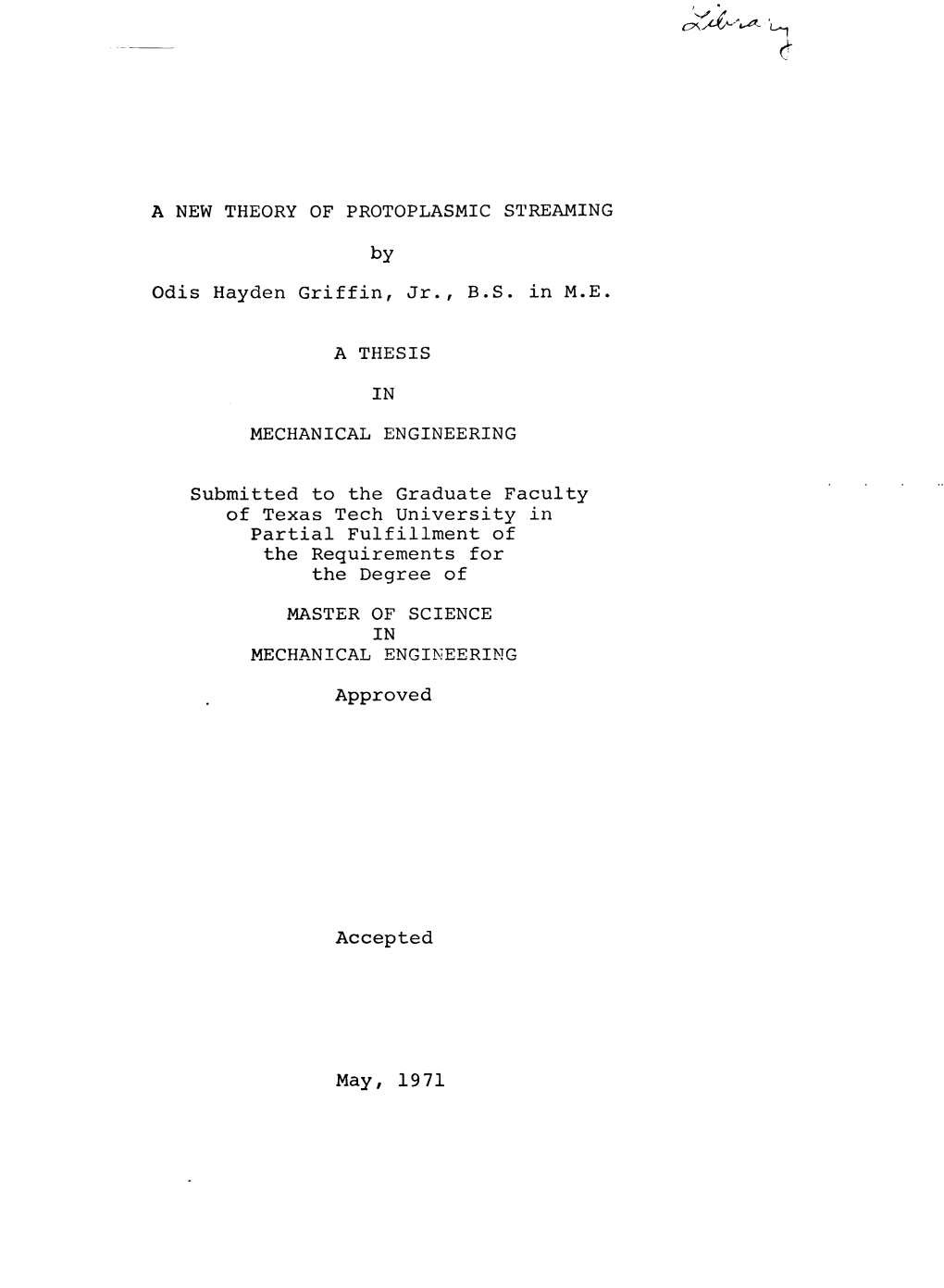
Load more
Recommended publications
-
A Viable Therapy Against Metastatic Breast Cancer
Graduate Theses, Dissertations, and Problem Reports 2017 Dual Targeting of the Mesenchymal and Amoeboid Pathways: A Viable Therapy against Metastatic Breast Cancer Brandon C. Jones Follow this and additional works at: https://researchrepository.wvu.edu/etd Recommended Citation Jones, Brandon C., "Dual Targeting of the Mesenchymal and Amoeboid Pathways: A Viable Therapy against Metastatic Breast Cancer" (2017). Graduate Theses, Dissertations, and Problem Reports. 5905. https://researchrepository.wvu.edu/etd/5905 This Dissertation is protected by copyright and/or related rights. It has been brought to you by the The Research Repository @ WVU with permission from the rights-holder(s). You are free to use this Dissertation in any way that is permitted by the copyright and related rights legislation that applies to your use. For other uses you must obtain permission from the rights-holder(s) directly, unless additional rights are indicated by a Creative Commons license in the record and/ or on the work itself. This Dissertation has been accepted for inclusion in WVU Graduate Theses, Dissertations, and Problem Reports collection by an authorized administrator of The Research Repository @ WVU. For more information, please contact [email protected]. Dual Targeting of the Mesenchymal and Amoeboid Pathways: A Viable Therapy against Metastatic Breast Cancer Brandon C. Jones Dissertation submitted to the School of Medicine at West Virginia University in partial fulfillment of the requirements for the degree of Doctor of Philosophy in Biochemistry & Molecular Biology Elena Pugacheva, Ph.D., Chair J. Michael Ruppert, M.D., Ph.D. Maxim Sokolov, Ph.D. Michael Schaller, Ph.D. Scott Weed, Ph.D. -
Some Considerations of Protoplasm
THE OHIO JOURNAL OF SCIENCE VOL. XXV MAY, 1925 No. 3 SOME CONSIDERATIONS OF PROTOPLASM. BRUCE FINK, Department of Botany, Miami University. One of the most fundamental problems in biological science is that which concerns protoplasm. Yet there is great diver- sity of opinion among biologists regarding what constitutes protoplasm and some doubt whether the term protoplasm is really worth retaining. In the present state of knowledge, protoplasm can not be defined in any terms of physical structure which will be accepted, without qualification, by a majority of botanists, and can only be defined somewhat more satis- factorily in terms of colloidal chemistry. Again, though chemi- cal definition is somewhat more certain than physical, this alone is far from satisfactory to those who think of cell con- tents in terms of microscopic structure. It is sometimes stated by certain biologists that proto- plasm is essentially alike in all organism. This may be true in the rough if we define in purely chemical terms; or if we content ourselves with the statement that protoplasm is the living substance of the cell, knowing not how much of the cell is alive, and, therefore, protoplasm. Turning to those very lowly organized plants, the bacteria, most of us will agree that the whole cell content, inclusive or exclusive of the vacuoles, composes the protoplasm. For higher fungi and for animals the situation is about the same, except that definite nuclei here replace the nuclear granules commonly supposed to exist in bacteria. Turning attention to higher green plants, we find that the cells are much more complex with respect to visible contents. -
The Cell-Theory: a Restatement, History, and Critique Part III
*57 The Cell-theory: A Restatement, History, and Critique Part III. The Cell as a Morphological Unit By JOHN R. BAKER (From the Department of Zoology and Comparative Anatomy, Oxford) SUMMARY A long time elapsed after the discovery of cells before they came to be generally regarded as morphological units. As a first step it was necessary to show that the cell-walls of plants were double and that cells could therefore be separated. The earliest advances in this direction were made by Treviranus (1805) and Link (1807). The idea of a cell was very imperfect, however, so long as attention was con- centrated on its wall. The first person who stated clearly that the cell-wall is not a necessary constituent was Leydig (1857). Subsequently the cell came to be regarded as a naked mass of protoplasm with a nucleus, and to this unit the name of protoplast was given. The true nature of the limiting membrane of the protoplast was discovered by Overton (1895). The plasmodesmata or connective strands that sometimes connect cells were prob- ably first seen by Hartig, in sieve-plates (1837). They are best regarded from the point of view of their functions in particular cases. They do not provide evidence for the view that the whole of a multicellular organism is basically a protoplasmic unit. Two or more nuclei in a continuous mass of protoplasm appear to have been seen for the first time in 1802, by Bauer. That an organism may consist wholly of a syn- cytium was discovered in i860, in the Mycetozoa. -
Intelligent Behaviors of Amoeboid Movement Based on Complex Dynamics of Soft Matter
REVIEW www.rsc.org/softmatter | Soft Matter Intelligent behaviors of amoeboid movement based on complex dynamics of soft matter Toshiyuki Nakagakiab and Robert D. Guyc Received 25th April 2007, Accepted 26th September 2007 First published as an Advance Article on the web 2nd November 2007 DOI: 10.1039/b706317m We review how soft matter is self-organized to perform information processing at the cell level by examining the model organism Physarum plasmodium. The amoeboid organism, Physarum polycephalum, in the class of true slime molds, exhibits the intelligent behavior of foraging in complex situations. When placed in a maze with food sources at two exits, the organism develops tubular structures with its body which connect the food sources along the shortest path so that the rates of nutrient absorption and intracellular communication are maximized. This intelligent behavior results from the organism’s control of a dynamic network through which mechanical and chemical information is transmitted. We review experimental studies that explore the development and adaptation of structures that make up the network. Recently a model of the dynamic network has been developed, and we review the formulation of this model and present some key results. The model captures the dynamics of existing networks, but it does not answer the question of how such networks form initially. To address the development of cell shape, we review existing mechanochemical models of the protoplasm of Physarum, present more general models of motile cells, and discuss how to adapt existing models to explore the development of intelligent networks in Physarum. 1. Introduction: intelligence at the cell level From a material science point of view, the cell is an exotic system in which nonliving materials act together to The cell is the elementary unit of all organisms. -
Bathybius Haeckelii and the Psychology of Scientific Discovery
NICOLAAS A. RUPKE BATHYBIUS HAECKELII AND THE PSYCHOLOGY OF SCIENTIFIC DISCOVERY THEORY INSTEAD OF OBSERVED DATA CONTROLLED THE LATE 19th CENTURY ‘DISCOVERY’ OF A PRIMITIVE FORM OF LIFE THE TRADITIONAL image of the scientist as an objective fact finder has become seriously tarnished by recent work in the history and philosophy of science. ’ It is argued that the growth of science is not always brought about by a reasoned debate based on objective evidence. Instead, scientific discovery seems to be controlled quite as much by certain psychological factors such as respect for a theoretical superstructure. The debate around T. S. Kuhn’s The Structure of Scientific Revolutions has brought similar iconoclastic aspects of scientific conduct to the attention of a cross section of the scholarly community.’ Without wanting to enter into the controversy generated by Kuhn’s book,3 this paper records one of the better examples from the annals of science to show how respect for a theoretical superstructure brought about a fictitious discovery. Specifically, it records how confidence in the heuristic value of evolutionary theory in the second half of the 19th century produced the discovery of a fictitious primitive form of life, called Bathybius, its sub-division into two genera, its reported occur- rence over vast regions of the ocean floor, its identification in the geologic record, and its wide acceptance in the life and earth sciences for the period of almost a decade. Background to the ‘Discovery’ Shortly after the publication of Darwin’s The Ongin of Species (1859), 1 See review paper by S. G. -
Membrane Potentials in Amoeba Proteus
J. Exp. Biol. (1966), 45, 251-267 251 With 10 text-figures Printed in Great Britain MEMBRANE POTENTIALS IN AMOEBA PROTEUS BY M. S. BINGLEY R.A.F. Institute of Aviation Medicine, Farnborough, Hants. {Received 16 February 1966) INTRODUCTION The possibility that the initiation of pseudopods together with the direction of cytoplasmic streaming may be induced by local depolarization of the membrane has been advanced from time to time for many years past. But is it difficult to find a direct statement to this effect in the literature. Amici (1818) suggested an electrical theory to account for streaming in plant cells and more recently Kitching (1961) considers the possibility of depolarization of the cell surface initiating contraction. When he discussed Hahnert's work (Hahnert, 1932) on the response of amoebae to electricity he pointed out that movement of the pseudopods towards the cathode may be an enhancement of 'local currents associated with local excitation'. It is one thing to put forward a hypothesis but another to obtain convincing measurements which are free from the suspicion of doubt and artifact. Membrane potentials were first seriously measured in Amoeba proteus by Telkes in 1931. Using large electrodes, she obtained low values of membrane potential but produced extremely valuable information on various depolarizing agents such as potassium and sodium chloride. Buchtal & Peterfi (1936) obtained low values of potential for A. proteus. Wolfson (1943) produced convincing values for membrane potential in Chaos chaos and, using electrodes of large diameter, he obtained potentials as high as workers using more modern micro- electrodes. More recently Riddle (1962) working on PeUomyxa caroHnensis recorded values of — 90 mV. -
Cytoplasmic Streaming and Microtubules in the Coenocytic Marine Alga, Caulerpa Prolifera
J. Cell Sci. 2, 465-472 (1967) 465 Printed in Great Britain CYTOPLASMIC STREAMING AND MICROTUBULES IN THE COENOCYTIC MARINE ALGA, CAULERPA PROLIFERA D. D. SABNIS AND W. P. JACOBS Biology Department, Princeton University, Princeton, N.J., U.S.A. SUMMARY Two distinct patterns of cytoplasmic streaming in the leaf of Caulerpa prolifera are described. Broad, longitudinally running, two-way streams are restricted to the endoplasm of one leaf surface. Also present are large numbers of narrow, two-way streams that coil helically through- out the endoplasm surrounding the central vacuole. Numerous unique bundles of aggregated, evenly spaced, oriented microtubules are distributed within the inner cytoplasm some distance from the cell wall. Cortical microtubules, as described for other plant material, have been only very infrequently encountered in Caulerpa and appear to be sparsely distributed. Apart from the prominent bundles of oriented microtubules, no other significant ultrastructural differences were noted between the stationary ectoplasm and streaming endoplasm. The possible cyto- skeletal role of the oriented microtubules in the development and maintenance of asymmetries in organ differentiation is discussed in relation to their direct or indirect influence on the directional migration of cytoplasmic components. INTRODUCTION Although there have been numerous reports of the occurrence of microtubular and microfibrillar elements in the cytoplasm of a variety of cell types, only a limited number of publications has described these structures in algal cells (Berkaloff, 1966; Nagai & Rebhun, 1966). The possible functions of cytoplasmic microtubules and microfilaments in the plant cell have been the subjects of some considerable con- jecture and controversy. Microtubules have been considered to play a role in the laying down of secondary walls in differentiating tissue (Wooding & Northcote, 1964), and cell-plate formation in dividing cells (Pickett-Heaps & Northcote, 1966). -
Emergence of Self-Organized Amoeboid Movement in a Multi-Agent Approximation of Physarum Polycephalum
Emergence of Self-Organized Amoeboid Movement in a Multi-Agent Approximation of Physarum polycephalum Jeff Jones and Andrew Adamatzky Centre for Unconventional Computing, University of the West of England, Coldharbour Lane, Bristol BS16 1QY, UK. [email protected] , [email protected] Abstract: The giant single-celled slime mould Physarum polycephalum exhibits complex morphological adaptation and amoeboid movement as it forages for food and may be seen as a minimal example of complex robotic behaviour. Swarm computation has previously been used to explore how spatio- temporal complexity can emerge from, and be distributed within, simple component parts and their interactions. Using a particle based swarm approach we explore the question of how to generate collective amoeboid movement from simple non-oscillatory component parts in a model of P. polycephalum . The model collective behaves as a cohesive and deformable virtual material, approximating the local coupling within the plasmodium matrix. The collective generates de-novo and complex oscillatory patterns from simple local interactions. The origin of this motor behaviour is distributed within the collective rendering is morphologically adaptive, amenable to external influence, and robust to simulated environmental insult. We show how to gain external influence over the collective movement by simulated chemo-attraction (pulling towards nutrient stimuli) and simulated light irradiation hazards ( pushing from stimuli). The amorphous and distributed properties of the collective are demonstrated by cleaving it into two independent entities and fusing two separate entities to form a single device, thus enabling it to traverse narrow, separate or tortuous paths. We conclude by summarising the contribution of the model to swarm based robotics and soft-bodied modular robotics and discuss the future potential of such material approaches to the field. -
Protozoa : Locomotion and Nutrition
Protozoa : locomotion and Nutrition Lecturer : P. V. Deokar Department of Zoology R.K.M.M. Ahmednagar Locomotion in Protozoa • The following points highlight the three main types of locomotion exhibited by protozoans. The types of locomotion are: • 1. Amoeboid Movement • 2. Flagellar Movement • 3. Ciliary Movement. Protozoans: Type of Locomotion # 1. Amoeboid Movement: • movement of the animal is made by the throwing of pseudopodium, called amoeboid movement • In the direction of movement of Amoeba a new pseudopodium is formed and the pseudopodium at the opposite side gradually disappears. • Types of pseudopodia: • According to form, structure and activity four different kinds of pseudopodia are recognised • These are: • (a) Lobopodium • (b) Filopodium • (c) Reticulopodium or Rhizopodium • (d) Axopodium or Actinopodium (a) Lobopodium [Gk. lobes = lobe; podium = foot]: • It is a short, finger or tongue-like projection which is accompanied by a flow of endoplasm and ectoplasm. • The pseudopodium is broad with rounded or blunt tips. • The ectoplasmmic area is distinctly clear, called the hyaline cap. • It is the characteristic of many amoebas such as Amoeba. (b) Filopodium [L.filo = a thread; podium = foot]: • The filopodium is a slender, thread-like or filamentous projection. • It is formed by the ectoplasm alone and without a hyaline cap. • The filaments are narrow and may be branched but do not anastomose, Filopodium is the characteristic in Filosea (e.g., Euglypha ). (c) Reticulopodium or Rhizopodium [L. reticulos = a net, podium = foot]: • Similar in structure to that of filopodium but the branches anastomose. • The numerous branched and anastomosed pseudopodia form a dense network, help primarily in capturing the prey and the secondary function is locomotion. -
Differential Organelle Movement on the Actin Cytoskeleton in Lily Pollen Tubes
Cell Motility and the Cytoskeleton (2007) Differential Organelle Movement on the Actin Cytoskeleton in Lily Pollen Tubes Alenka Lovy-Wheeler,1 Luis Ca´rdenas,2 Joseph G. Kunkel,1 and Peter K. Hepler1* 1Department of Biology and Plant Biology Graduate Program, Morrill Science Center III, University of Massachusetts, Amherst, Massachusetts 2Departamento de Biologı´a Molecular de Plantas, Instituto de Biotechnologı´a, Cuernavaca, Morelos, Me´xico We have examined the arrangement and movement of three major compart- ments, the endoplasmic reticulum (ER), mitochondria, and the vacuole during oscillatory, polarized growth in lily pollen tubes. These movements are de- pendent on the actin cytoskeleton, because they are strongly perturbed by the anti-microfilament drug, latrunculin-B, and unaffected by the anti-microtubule agent, oryzalin. The ER, which has been labeled with mGFP5-HDEL or cyto- chalasin D tetramethylrhodamine, displays an oscillatory motion in the pollen tube apex. First it moves apically in the cortical region, presumably along the cortical actin fringe, and then periodically folds inward creating a platform that transects the apical domain in a plate-like structure. Finally, the ER reverses its direction and moves basipetally through the central core of the pollen tube. When subjected to cross-correlation analysis, the formation of the platform precedes maximal growth rates by an average of 3 s (35–408). Mitochondria, labeled with Mitotracker Green, are enriched in the subapical region, and their movement closely resembles that of the ER. The vacuole, labeled with car- boxy-dichlorofluorescein diacetate, consists of thin tubules arranged longitudi- nally in a reticulate network, which undergoes active motion. -
On the Physiology of Amoeboid Movement
ON THE PHYSIOLOGY OF AMOEBOID MOVEMENT. IV.—THE ACTION OF MAGNESIUM. BY C. F. A. PANTIN. {The Marine Biological Laboratory, Plymouth?) (Received January 6th, 1926.) CONTENTS 1. Maintenance of the cell-surface . 297 6. The action of Ce'" in the pres- 2. The action of barium . 300 ence of Ca" .... 306 3. The action of cerium . 301 4. Action of Mg" in the presence 7. Discussion 306 of Ca" 302 8. Summary 310 5. Interaction of the ions of sea- 9. References 311 water 303 THE previous paper of this series (Pantin, 1926) described the action of certain ions upon a species of marine amoeba, and especially the relation of calcium to amoeboid movement. The same paper detailed the method of preparing isotonic salt solutions and of determining their effect on amoeboid movement. The essential feature is that the average velocity is taken as a measure of the effect of a solution upon the power of movement, apart from other effects produced upon the cell. Unless otherwise stated, the CH of the solutions was maintained at /H 7.0 to 7.2. 1. Maintenance of the cell-surface. It was shown previously that whereas cytolysis occurred rapidly in pure isotonic NaCl or KCl (at about />H 7), amoebae remained alive for a longer time in isotonic CaCl= and MgClj. Movement was inhibited in these solutions, but reversibly, for, provided immersion had been brief, recovery occurred on return to natural sea-water. In mixtures of two salts, it was found that movement only occurred if Ca were 297 C. F. A. -
Hydrodynamic Flow in the Cytoplasm of Plant Cells
Journal of Microscopy, Vol. 231, Pt 2 2008, pp. 274–283 Received 14 June 2008; accepted 28 March 2008 Hydrodynamic flow in the cytoplasm of plant cells A. ESSELING-OZDOBA∗,‡,D.HOUTMAN†, A.A.M. VAN LAMMEREN∗, E. EISER† & A.M.C. EMONS∗ ∗Laboratory of Plant Cell Biology, Department of Plant Sciences, Wageningen University, Arboretumlaan 4, 6703 BD Wageningen, The Netherlands †van’t Hoff Institute for Molecular Sciences (HIMS), Universiteit van Amsterdam, Nieuwe Achtergracht 166, 1018 WV Amsterdam, The Netherlands ‡Current address: Department of Tumor Immunology, Nijmegen Centre for Molecular Life Sciences (NCMLS), Geert Grooteplein zuid 28, 6500 HB Nijmegen, The Netherlands Key words. Cytoplasmic streaming, FRAP, GFP, hydrodynamic flow, lipid vesicles, micro-injection, tobacco BY-2 suspension cultured cells, Tradescantia virginiana. Summary that synthetic lipid (DOPG) vesicles and ‘stealth’ vesicles with PEG phospholipids moved in the cytoplasm. Plant cells show myosin-driven organelle movement, called cytoplasmicstreaming.Solublemolecules,suchasmetabolites do not move with motor proteins but by diffusion. However, is all of this streaming active motor-driven organelle transport? Introduction Our recent simulation study (Houtman et al., 2007) shows The cytoplasm of eukaryotic cells consists of all cell material that active transport of organelles gives rise to a drag in the between the nucleus and the plasma membrane and cytosol, setting up a hydrodynamic flow, which contributes to contains membrane-bounded structures, organelles, which a fast distribution of proteins and nutrients in plant cells. Here, are embedded in the cytosol consisting of water, salts and we show experimentally that actively transported organelles organic molecules, including sugars, proteins and many produce hydrodynamic flow that significantly contributes to enzymes that catalyze reactions.