Temperature-Insensitive Semiconductor Quantum Dot Laser Levon V
Total Page:16
File Type:pdf, Size:1020Kb
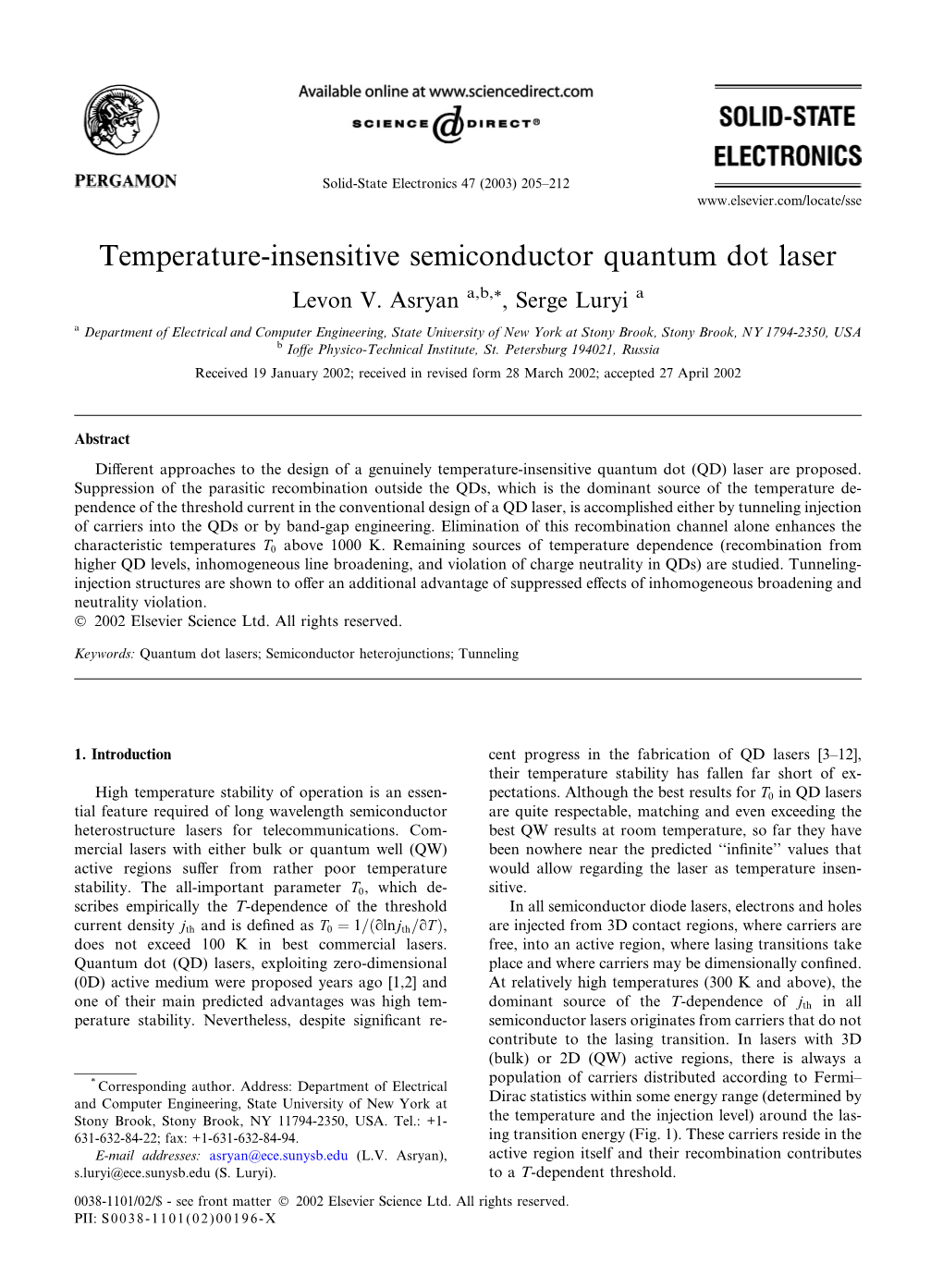
Load more
Recommended publications
-
Monday, 08:00–10:00 CLEO: QELS-Fundamental Science
07:00–18:00 Registration, Concourse Level Executive Ballroom Executive Ballroom Executive Ballroom Executive Ballroom 210A 210B 210C 210D CLEO: QELS-Fundamental Science 08:00–10:00 08:00–10:00 08:00–10:00 08:00–10:00 FM1A • Quantum FM1B • Topological Photonics I FM1C • Novel Phenomena in FM1D • Coherent Phenomena Optomechanics & Transduction Presider: To Be Announced Classical Nano-Optics in Coupled Resonator Networks Monday, 08:00–10:00 Monday, Presider: Gabriel Molina Terriza; Presider: Mo Mojahedi; Univ. of Presider: To Be Announced Centro de Fisica de Materiales, Toronto, USA Spain FM1A.1 • 08:00 FM1B.1 • 08:00 FM1C.1 • 08:00 FM1D.1 • 08:00 Invited Ultralow Dissipation Mechanical Resona- Spin-Preserving Chiral Photonic Crystal Brightness Theorems for Nanophoton- Solving Hard Computational Problems with 1,2 1 1 tors for Quantum Optomechanics, Nils Mirror, Behrooz Semnani , Jeremy Flan- ics, Hanwen Zhang , Chia Wei Hsu , Owen Coupled Lasers, Nir Davidson1; 1Weizmann 1 1 2 2 2 1 1 Johan Engelsen , Sergey A. Fedorov , Amir nery , Zhenghao Ding , Rubayet Al Maruf , Miller ; Yale Univ., USA. We present nano- Inst. of Science, Israel. We present a new a 1 1 2,1 1 H. Ghadimi , Mohammad J. Bereyhi , Alberto Michal Bajcsy ; ECE, Univ. of Waterloo, photonic ‘’brightness theorems’’, a set of new system of coupled lasers in a modified 1 1 2 2 Beccari , Ryan Schilling , Dalziel J. Wilson , Canada; Inst. for Quantum Computing, power-concentration bounds that generalize degenerate cavity that is used to solve dif- 1 1 Tobias J. Kippenberg ; Ecole Polytechnique Canada. We report on experimental realiza- their ray-optical counterparts, and motivate ficult computational tasks. -
UC Santa Barbara Dissertation Template
UC Santa Barbara UC Santa Barbara Electronic Theses and Dissertations Title Quantum Dot Lasers for Silicon Photonics Permalink https://escholarship.org/uc/item/8p01r83d Author Norman, Justin Publication Date 2018 Peer reviewed|Thesis/dissertation eScholarship.org Powered by the California Digital Library University of California UNIVERSITY OF CALIFORNIA Santa Barbara Quantum Dot Lasers for Silicon Photonics A dissertation submitted in partial satisfaction of the requirements for the degree Doctor of Philosophy in Materials by Justin Colby Norman Committee in charge: Professor John Bowers, Co-Chair Professor Arthur Gossard, Co-Chair Professor Chris Palmstrøm Professor Dirk Bouwmeester December 2018 The dissertation of Justin Colby Norman is approved. ____________________________________________ Professor Dirk Bouwmeester ____________________________________________ Professor Chris Palmstrøm Professor Arthur Gossard, Committee Co-Chair ____________________________________________ Professor John Bowers, Committee Co-Chair December 2018 Quantum Dot Lasers for Silicon Photonics Copyright © 2018 by Justin Colby Norman iii Dedicated to April Norman iv ACKNOWLEDGEMENTS If a thesis were like a journal article and all contributors given credit in the author list, then my title page would exceed entire length of this body of work. Pursuing a Ph.D. is not a simple endeavor, and its pursuit begins long before a student begins their journey in graduate school. To do proper justice to everyone in my life who contributed to my efforts would not be possible in the limited scope of this document, but I will attempt to acknowledge the key individuals who got me here, helped along the way in my scientific endeavors, and who helped me maintain the tenuous grip on sanity inherent to graduate studies. -
Symposium on Undergraduate Research
SYMPOSIUM ON UNDERGRADUATE RESEARCH Division of Laser Science of A.P.S - LS XXXIV - 17 September 2018.- Washington DC PARTICIPANTS’ LUNCHEON - Ballroom East - 12:00 The participants' luncheon will bring together the Symposium students and distinguished laser scientists. Sandwich lunches will be provided for participants and invited guests only. REMINDER: Group Photo Break 3:55 PM - PLEASE assemble at the designated place!!! POSTER SESSION - International Ballroom East - 1:00 Session LM4G (poster) 1:00 - 3:55 PM, Ballroom East – Dr. Keith Stein, Bethel Univ., Presider LM4G - 1 Using the Instantaneous Velocity of a Brownian Particle in an Optical Tweezer to Measure Changes in Mass. Gabriel H. Alvarez1, Julia E. Orenstein2, Lichung Ha2, Diney S. Ether Jr.2, and Mark G. Raizen2. 1) Stanford Univ., Stanford, CA 94305, 2) Univ. of Texas, Austin, TX 78712. Using a fast detector to observe the light deflected by silica mi- crospheres trapped in an optical tweezer, we obtain positional information from which instantaneous velocities are calcu- lated and fit to the Maxwell-Boltzmann distribution to extract mass. We plan to use this technology to characterize the onset of heterogeneous ice nucleation. LM4G - 2 Optical Tweezing Experiments with Dielectric Microbeads Willa Dworschack1,2, Chiu Yin Lee1, Perri Zilberman1, Martin Cohen1, Harold Metcalf 11) Stony Brook Univ., Stony Brook, NY 11794, 2) Lawrence Univ., Appleton, WI 54911 Optical tweezing utilizes the momentum carried by light to manipulate micro-scale objects. We designed and constructed an optical tweezing apparatus that enabled precision control of dielectric microbeads using a He-Ne laser and an inverted microscope. Its piconewton force capabilities were demonstrated. -
III-NITRIDE SELF-ASSEMBLED QUANTUM DOT LIGHT EMITTING DIODES and LASERS by Animesh Banerjee a Dissertation Submitted in Partial
III-NITRIDE SELF-ASSEMBLED QUANTUM DOT LIGHT EMITTING DIODES AND LASERS by Animesh Banerjee A dissertation submitted in partial fulfillment of the requirements for the degree of Doctor of Philosophy (Electrical Engineering) in The University of Michigan 2014 Doctoral Committee: Professor Pallab K. Bhattacharya, Chair Associate Professor Pei-Cheng Ku Professor Joanna Mirecki-Millunchick Professor Jamie D. Phillips Animesh Banerjee © 2014 All Rights Reserved To my parents who have always stood by me, and have showered their blessings, unconditional love and unwavering support ii ACKNOWLEDGMENT I would like to express my sincere gratitude to my advisor Prof. Pallab Bhattacharya for his continuous support and motivation, his immense knowledge, enthusiasm and patience powering me along the way during my PhD years. He is a great motivator and educator. He was always available to discuss my research and any technical problem that I encountered. His sheer persistence and determination in solving any problem is something I really admire and aspire to pursue for the rest of my life. It has been truly an honor to work with Prof. Bhattacharya. I am also grateful to my committee memebers, Prof. Pei-Cheng Ku, Prof. Jamie Phillips, and Prof. Joanna Mirecki-Millunchick, for their time, insightful comments, and valuable suggestions. I would like to specially thank Prof. Millunchick for her valuable discussions and inputs during our collaborative endeavor. I am thankful to Dr. Meng Zhang and Dr. Wei Guo, my mentors in this group for getting me aquainted with epitaxial growth, fabrication and characterization. I would like to thank Dr. Junseok Heo for guiding me in my first research project here. -
Solid State Laser
SOLID STATE LASER Edited by Amin H. Al-Khursan Solid State Laser Edited by Amin H. Al-Khursan Published by InTech Janeza Trdine 9, 51000 Rijeka, Croatia Copyright © 2012 InTech All chapters are Open Access distributed under the Creative Commons Attribution 3.0 license, which allows users to download, copy and build upon published articles even for commercial purposes, as long as the author and publisher are properly credited, which ensures maximum dissemination and a wider impact of our publications. After this work has been published by InTech, authors have the right to republish it, in whole or part, in any publication of which they are the author, and to make other personal use of the work. Any republication, referencing or personal use of the work must explicitly identify the original source. As for readers, this license allows users to download, copy and build upon published chapters even for commercial purposes, as long as the author and publisher are properly credited, which ensures maximum dissemination and a wider impact of our publications. Notice Statements and opinions expressed in the chapters are these of the individual contributors and not necessarily those of the editors or publisher. No responsibility is accepted for the accuracy of information contained in the published chapters. The publisher assumes no responsibility for any damage or injury to persons or property arising out of the use of any materials, instructions, methods or ideas contained in the book. Publishing Process Manager Iva Simcic Technical Editor Teodora Smiljanic Cover Designer InTech Design Team First published February, 2012 Printed in Croatia A free online edition of this book is available at www.intechopen.com Additional hard copies can be obtained from [email protected] Solid State Laser, Edited by Amin H. -
Zhang Gsas.Harvard 0084L 10989.Pdf (11.58Mb)
Manipulating Light on Wavelength Scale The Harvard community has made this article openly available. Please share how this access benefits you. Your story matters Citation Zhang, Yinan. 2012. Manipulating Light on Wavelength Scale. Doctoral dissertation, Harvard University. Citable link http://nrs.harvard.edu/urn-3:HUL.InstRepos:11051175 Terms of Use This article was downloaded from Harvard University’s DASH repository, and is made available under the terms and conditions applicable to Other Posted Material, as set forth at http:// nrs.harvard.edu/urn-3:HUL.InstRepos:dash.current.terms-of- use#LAA Manipulating Light on Wavelength Scale Y Z T S E A S D P E E H U C, M D © - Y Z A . esis advisor: Marko Loncar Yinan Zhang Manipulating Light on Wavelength Scale A Light, at the length-scale on the order of its wavelength, does not simply behave as “light ray”, but instead diffracts, scaers, and interferes with itself, as governed by Maxwell’s equations. A profound understanding of the underlying physics has inspired the emergence of a new frontier of materials and devices in the past few decades. is thesis explores the concepts and approaches for manipulating light at the wavelength-scale in a variety of topics, including anti-reective coatings, on- chip silicon photonics, optical microcavities and nanolasers, microwave particle accelerators, and optical nonlinearities. In Chapter , an optimal tapered prole that maximizes light transmission be- tween two media with different refractive indices is derived from analytical theory and numerical modeling. A broadband wide-angle anti-reective coating at the air/silicon interface is designed for the application of photovoltaics. -
Front Cover CS V6mg.Indd 1 31/08/2017 16:10 Untitled-1 1 26/07/2017 09:21 Viewpoint by Dr Richard Stevenson, Editor
Volume 23 Issue 6 August / September 2017 @compoundsemi www.compoundsemiconductor.net Lighting up silicon with InAs quantum dots MACOM Enabling breakthroughs in optical bandwidth density Novel cooling aid high-power laser diodes Easing the use of GaN power electronics An abundance of key elements for chipmakers Ultraviolet LEDs take aim at disinfection News Review, News Analysis, Features, Research Review, and much more... inside Free Weekly E News round up go to: www.compoundsemiconductor.net Front Cover CS v6MG.indd 1 31/08/2017 16:10 Untitled-1 1 26/07/2017 09:21 Viewpoint By Dr Richard Stevenson, Editor Telling our story POPULAR SCIENCES BOOKS aim to educate the layman. By better is to tell the story the way Johnstone does. It begins with eradicating equations, offering analogies and telling a story with a meeting of visionaries that plot out the rate of improvement a human touch, Joe Public can be entertained while learning in these devices, see where they are destined to go, and put the basics of a subject. plans in place to make this happen. Instrumental in the success is the backing by government, which uses its money very But that’s not the only benefi t of these books. They can be read effectively, alongside the introduction of standards that quash by experts, so that when they are at social gatherings and are the availability of inferior bulbs. asked what they do for a living, they can draw on what’s been written to give an answer that’s engaging and understandable. Read this book and you will also be able to wax lyrical about the benefi ts of LEDs in It is for that reason that I recommend Bob Johnstone’s new agriculture, and how the tuning of the book L.E.D. -
Techniques for High-Speed Direct Modulation of Quantum Dot Lasers Yan Li
University of New Mexico UNM Digital Repository Optical Science and Engineering ETDs Engineering ETDs 7-21-2008 Techniques for high-speed direct modulation of quantum dot lasers Yan Li Follow this and additional works at: https://digitalrepository.unm.edu/ose_etds Recommended Citation Li, Yan. "Techniques for high-speed direct modulation of quantum dot lasers." (2008). https://digitalrepository.unm.edu/ose_etds/ 17 This Dissertation is brought to you for free and open access by the Engineering ETDs at UNM Digital Repository. It has been accepted for inclusion in Optical Science and Engineering ETDs by an authorized administrator of UNM Digital Repository. For more information, please contact [email protected]. Yan Li Candidate Department of Electrical and Computer Engineering Department This dissertation is approved, and it is acceptable in quality and form for publication on microfilm: Approved by the Dissertation Committee: , Chairperson Accepted: Dean, Graduate School Date TECHNIQUES FOR HIGH-SPEED DIRECT MODULATION OF QUANTUM DOT LASERS BY YAN LI M.S. Optics, Sichuan University, 2000 DISSERTATION Submitted in Partial Fulfillment of the Requirements for the Degree of Doctor of Philosophy Optical Science and Engineering The University of New Mexico Albuquerque, New Mexico May, 2008 ACKNOWLEDGEMENTS My deepest gratitude is to my advisor, Prof. Luke F. Lester. I was fortunate to have his support to continue my research in the semiconductor laser area. His guidance, perspective and encouragement are always a major driving force for me to fulfill my dissertation and will benefit my future career. I really appreciate his infinite understanding and patience during the last four years. I also would like to thank Prof. -
The Study of Coupling in Ingaas Quantum Rings Grown by Droplet Epitaxy
The Study of Coupling in InGaAs Quantum Rings Grown by Droplet Epitaxy A thesis presented to the faculty of the College of Arts and Sciences of Ohio University In partial fulfillment of the requirements for the degree Master of Science Samar M. Alsolamy May 2013 © 2013 Samar M. Alsolamy. All Rights Reserved. 2 This thesis titled The Study of Coupling in InGaAs Quantum Rings Grown by Droplet Epitaxy by SAMAR M. ALSOLAMY has been approved for the Department of Physics and Astronomy and the College of Arts and Sciences by Eric A. Stinaff Associate Professor of Physics and Astronomy Robert Frank Dean, College of Arts and Sciences 3 ABSTRACT ALSOLAMY, SAMAR, M., M.S., May 2013, Physics and Astronomy The Study of Coupling in InGaAs Quantum Rings Grown by Droplet Epitaxy Director ofThesis: Eric A. Stinaff The use of metal droplet epitaxy may provide a novel method of growing laterally coupled nanostructures. We will present optical studies of InAs/GaAs nanostructures which result in twin quantum dots (QD) formed on a single quantum ring (QR). Previous studies have investigated the coupling between vertically grown quantum dot pairs. In this thesis, we have used photoluminescence (PL) and photoluminescence excitation (PLE) to examine the possibility of energy transfer and coupling between quantum dot pairs in a single InGaAs quantum ring grown by droplet epitaxy. Power dependent photoluminescence spectra reveal a few peaks at low power, which are identified with emission from the ground state of the individual dots. As the power is increased we observe multi-exciton and excited state emission. We then perform PLE, tuning the excitation laser energy continuously from the high energy ring emission down to the individual dot states. -
A Solution-Processed 1.53 Μm Quantum Dot Laser with Temperature
A solution-processed 1.53 μm quantum dot laser with temperature-invariant emission wavelength S. Hoogland, V. Sukhovatkin, I. Howard, S. Cauchi, L. Levina, E. H. Sargent The Edward S. Rogers Sr. Department of Electrical and Computer Engineering University of Toronto Toronto, ON, Canada, M5S 3G4 [email protected]; [email protected] Abstract: Sources of coherent, monochromatic short-wavelength infrared (1-2 μm) light are essential in telecommunications, biomedical diagnosis, and optical sensing. Today’s semiconductor lasers are made by epitaxial growth on a lattice-matched single-crystal substrate. This strategy is incompatible with direct growth on silicon. Colloidal quantum dots synthesized in solution can, in contrast, be coated onto any surface. Here we show a 1.53 μm laser fabricated using a remarkably simple process: dipping a glass capillary into a colloidal suspension of semiconductor quantum dots. We developed the procedures to produce a smooth, low-scattering-loss film inside the capillary, resulting in a whispering gallery mode laser with a well-defined threshold. While there exist three prior reports of optical gain in infrared-emitting colloidal quantum dots [1, 2, 3], this work represents the first report of an infrared laser made using solution processing. We also report dλmax/dT, the temperature-sensitivity of lasing wavelength, of 0.03 nm/K, the lowest ever reported in a colloidal quantum dot system and 10 times lower than in traditional semiconductor quantum wells. ©2006 Optical Society of America OCIS codes: (140.5960) Semiconductor lasers; (160.3380) Laser materials; (999.999) Nanocrystal quantum dots. References and links 1. R. -
Foucher-Etal-IEEE-PJ-2018-Flexible-Glass-Hybridized-Colloidal
IEEE Photonics Journal Flexible Glass Hybridized Colloidal Quantum Dots Flexible Glass Hybridized Colloidal Quantum Dots for Gb/s Visible Light Communications Caroline Foucher,1 Mohamed Islim Sufyan ,2 Student Member, IEEE, Benoit Jack Eloi Guilhabert ,1 Stefan Videv,2 Sujan Rajbhandari ,3∗ Member, IEEE,ArielGomezDiaz ,3 Hyunchae Chun ,3 Dimali A. Vithanage,4 Graham A. Turnbull ,4 Senior Member, IEEE,IforD.W.Samuel,4 Grahame Faulkner,3 Dominic C. O’Brien,3 Harald Haas ,2 Nicolas Laurand ,1 Member, IEEE, and Martin D. Dawson ,1 Fellow, IEEE 1Institute of Photonics, Department of Physics, SUPA, University of Strathclyde, Glasgow G1 1RD, U.K. 2LiFi Research and Development Centre, Institute for Digital Communications, University of Edinburgh, Edinburgh EH9 3JL, U.K. 3Oxford Communications Research Group, Department of Engineering Science, University of Oxford, Oxford OX1 3PJ, U.K. 4Organic Semiconductor Centre, SUPA, School of Physics and Astronomy, University of St Andrews, St Andrews KY16 9SS, U.K. DOI:10.1109/JPHOT.2018.2792700 This work is licensed under a Creative Commons Attribution 3.0 License. For more information, see http://creativecommons.org/licenses/by/3.0/ Manuscript received December 20, 2017; revised January 8, 2018; accepted January 9, 2018. Date of publication January 12, 2018; date of current version February 21, 2018. This work was sup- ported by the EPSRC through the Program grant ‘Ultra-parallel visible light communications (UP-VLC)’ (EP/K00042X/1). Corresponding author: Nicolas Laurand (email: [email protected]). *S. Rajbhandari is now with the Centre for Mobility & Transport, School of Computing, Engineering and Mathematics, Coventry University, Coventry CV1 5FB, U.K. -
Reflection Insensitive Quantum Dot Lasers Grown on Silicon Substrates
Reflection Insensitive Quantum Dot Lasers Grown on Silicon Substrates John Bowers Art Gossard, Songtao Liu, Justin Norman, Yating Wan, Chen Zhang, Robert Zheng Bob Herrick (Intel) Weng Chao (Sandia) Frederic Grillot (U. Paris) November 1, 2019 Team Project Team ‣ Silicon Photonics Research Group at UCSB – State of the art equipment for the characterization and packaging of a wide range of semiconductor devices and optical communication systems ‣ UCSB Nanofab – >10,000 ft2 of Class 100 and 1000 cleanroom space – Optical lithographic capability to 200 nm ‣ UCSB Growth Facilities – 30 years of pioneering MBE research – 9 MBE systems with two dedicated to III-Vs ‣ California Nanosystems Institute – Advanced material characterization tools – ECCI, TEM, AFM, XRD, SIMS, atom probe 1 Team Collaborators ‣ Frederic Grillot, ParisTech – Laser dynamics, feedback stability ‣ Robert Herrick, Intel Corp. – Laser reliability and aging ‣ Matteo Meneghini, Univ. of Padova – Laser reliability and aging ‣ Weng Chow, Sandia – Laser theory for linewidth enhancement factor and mode- locking 2 Project Objectives Achieve High Performance Epitaxial Lasers on Silicon ‣ Leverage silicon manufacturing infrastructure – Photonics in CMOS foundries – Economical, high integration density photonic circuits ‣ Indirect bandgap necessitates III-Vs for lasers – Heterogeneous integration: high cost, limited scalability – Epitaxial growth: low cost, scalable with Si wafer size 50 mm InP wafer 3 Why Quantum dot lasers? Lower threshold Higher temperature operation (220C) Lower