Determination of Shadowing on the Lunar Surface Using a Lunar-Celestial Equatorial Coordinate System
Total Page:16
File Type:pdf, Size:1020Kb
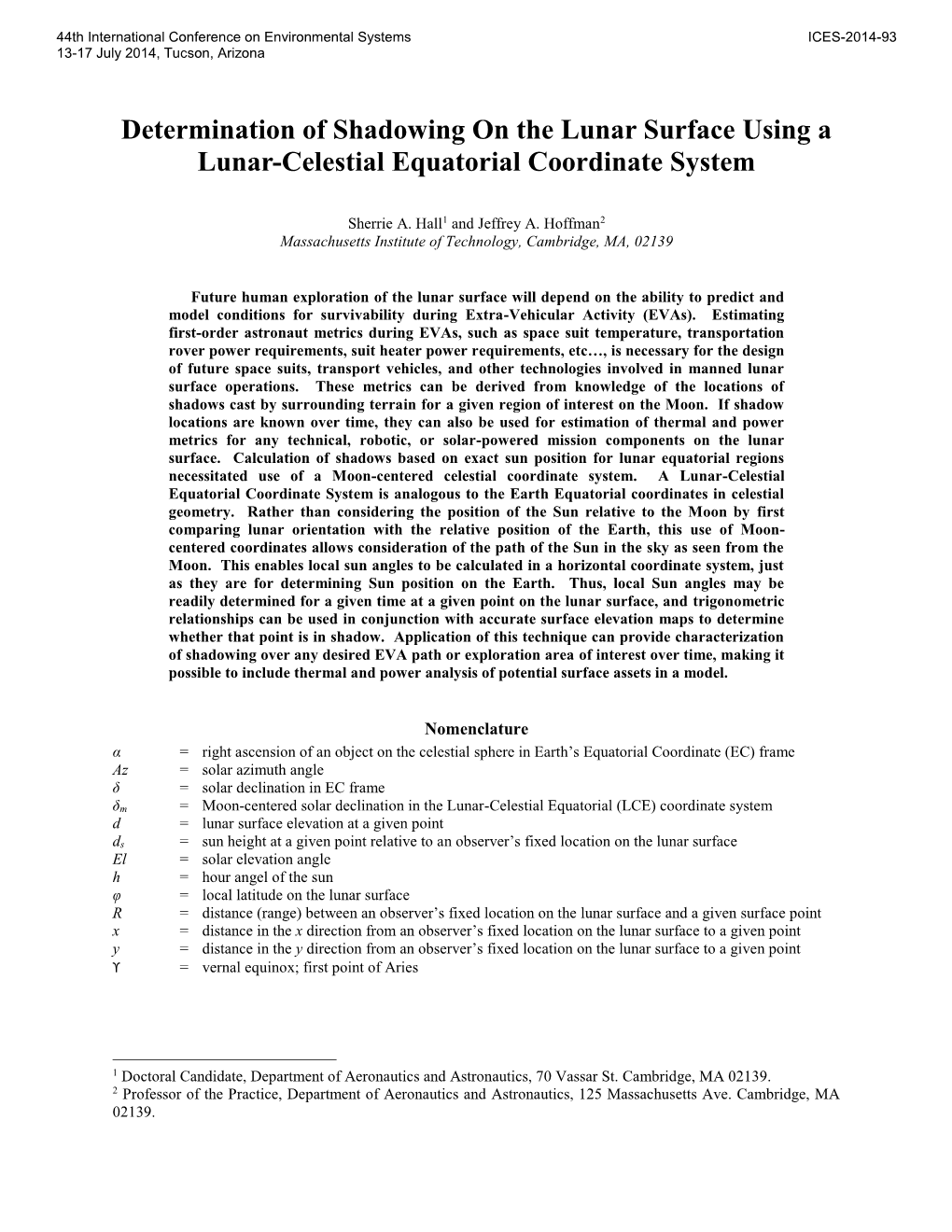
Load more
Recommended publications
-
Planet Positions: 1 Planet Positions
Planet Positions: 1 Planet Positions As the planets orbit the Sun, they move around the celestial sphere, staying close to the plane of the ecliptic. As seen from the Earth, the angle between the Sun and a planet -- called the elongation -- constantly changes. We can identify a few special configurations of the planets -- those positions where the elongation is particularly noteworthy. The inferior planets -- those which orbit closer INFERIOR PLANETS to the Sun than Earth does -- have configurations as shown: SC At both superior conjunction (SC) and inferior conjunction (IC), the planet is in line with the Earth and Sun and has an elongation of 0°. At greatest elongation, the planet reaches its IC maximum separation from the Sun, a value GEE GWE dependent on the size of the planet's orbit. At greatest eastern elongation (GEE), the planet lies east of the Sun and trails it across the sky, while at greatest western elongation (GWE), the planet lies west of the Sun, leading it across the sky. Best viewing for inferior planets is generally at greatest elongation, when the planet is as far from SUPERIOR PLANETS the Sun as it can get and thus in the darkest sky possible. C The superior planets -- those orbiting outside of Earth's orbit -- have configurations as shown: A planet at conjunction (C) is lined up with the Sun and has an elongation of 0°, while a planet at opposition (O) lies in the opposite direction from the Sun, at an elongation of 180°. EQ WQ Planets at quadrature have elongations of 90°. -
Sun Tool Options
Sun Study Tools Sophomore Architecture Studio: Lighting Lecture 1: • Introduction to Daylight (part 1) • Survey of the Color Spectrum • Making Light • Controlling Light Lecture 2: • Daylight (part 2) • Design Tools to study Solar Design • Architectural Applications Lecture 3: • Light in Architecture • Lighting Design Strategies Sun Tool Options 1. Paper and Pencil 2. Build a Model 3. Use a Computer The first step to any of these options is to define…. Where is the site? 1 2 Sun Study Tools North Latitude and Longitude South Longitude Latitude Longitude Sun Study Tools North America Latitude 3 United States e ud tit La Sun Study Tools New York 72w 44n 42n Site Location The site location is specified by a latitude l and a longitude L. Latitudes and longitudes may be found in any standard atlas or almanac. Chart shows the latitudes and longitudes of some North American cities. Conventions used in expressing latitudes are: Positive = northern hemisphere Negative = southern hemisphere Conventions used in expressing longitudes are: Positive = west of prime meridian (Greenwich, United Kingdom) Latitude and Longitude of Some North American Cities Negative = east of prime meridian 4 Sun Study Tools Solar Path Suns Position The position of the sun is specified by the solar altitude and solar azimuth and is a function of site latitude, solar time, and solar declination. 5 Sun Study Tools Suns Position The rotation of the earth about its axis, as well as its revolution about the sun, produces an apparent motion of the sun with respect to any point on the altitude earth's surface. The position of the sun with respect to such a point is expressed in terms of two angles: azimuth The sun's position in terms of solar altitude (a ) and azimuth (a ) solar azimuth, which is the t s with respect to the cardinal points of the compass. -
Equatorial and Cartesian Coordinates • Consider the Unit Sphere (“Unit”: I.E
Coordinate Transforms Equatorial and Cartesian Coordinates • Consider the unit sphere (“unit”: i.e. declination the distance from the center of the (δ) sphere to its surface is r = 1) • Then the equatorial coordinates Equator can be transformed into Cartesian coordinates: right ascension (α) – x = cos(α) cos(δ) – y = sin(α) cos(δ) z x – z = sin(δ) y • It can be much easier to use Cartesian coordinates for some manipulations of geometry in the sky Equatorial and Cartesian Coordinates • Consider the unit sphere (“unit”: i.e. the distance y x = Rcosα from the center of the y = Rsinα α R sphere to its surface is r = 1) x Right • Then the equatorial Ascension (α) coordinates can be transformed into Cartesian coordinates: declination (δ) – x = cos(α)cos(δ) z r = 1 – y = sin(α)cos(δ) δ R = rcosδ R – z = sin(δ) z = rsinδ Precession • Because the Earth is not a perfect sphere, it wobbles as it spins around its axis • This effect is known as precession • The equatorial coordinate system relies on the idea that the Earth rotates such that only Right Ascension, and not declination, is a time-dependent coordinate The effects of Precession • Currently, the star Polaris is the North Star (it lies roughly above the Earth’s North Pole at δ = 90oN) • But, over the course of about 26,000 years a variety of different points in the sky will truly be at δ = 90oN • The declination coordinate is time-dependent albeit on very long timescales • A precise astronomical coordinate system must account for this effect Equatorial coordinates and equinoxes • To account -
II. Causes of Tides III. Tidal Variations IV. Lunar Day and Frequency of Tides V
Tides I. What are Tides? II. Causes of Tides III. Tidal Variations IV. Lunar Day and Frequency of Tides V. Monitoring Tides Wikimedia FoxyOrange [CC BY-SA 3.0 Tides are not explicitly included in the NGSS PerFormance Expectations. From the NGSS Framework (M.S. Space Science): “There is a strong emphasis on a systems approach, using models oF the solar system to explain astronomical and other observations oF the cyclic patterns oF eclipses, tides, and seasons.” From the NGSS Crosscutting Concepts: Observed patterns in nature guide organization and classiFication and prompt questions about relationships and causes underlying them. For Elementary School: • Similarities and diFFerences in patterns can be used to sort, classiFy, communicate and analyze simple rates oF change For natural phenomena and designed products. • Patterns oF change can be used to make predictions • Patterns can be used as evidence to support an explanation. For Middle School: • Graphs, charts, and images can be used to identiFy patterns in data. • Patterns can be used to identiFy cause-and-eFFect relationships. The topic oF tides have an important connection to global change since spring tides and king tides are causing coastal Flooding as sea level has been rising. I. What are Tides? Tides are one oF the most reliable phenomena on Earth - they occur on a regular and predictable cycle. Along with death and taxes, tides are a certainty oF liFe. Tides are apparent changes in local sea level that are the result of long-period waves that move through the oceans. Photos oF low and high tide on the coast oF the Bay oF Fundy in Canada. -
Building Structures on the Moon and Mars: Engineering Challenges and Structural Design Parameters for Proposed Habitats
Building Structures on the Moon and Mars: Engineering Challenges and Structural Design Parameters for Proposed Habitats Ramesh B. Malla, Ph.D., F. ASCE, A.F. AIAA Professor Department of Civil and Environmental Engineering University of Connecticut, Storrs, CT 06269 (E-Mail: [email protected]) Presented at the Breakout Panel Session- Theme C - Habitats (Preparation and Architecture) RETH Workshop- Grand Challenges and Key Research Questions to Achieve Resilient Long-Term Extraterrestrial Habitats Purdue University; October 22-23, 2018 What is Structural Resiliency? Characterized by four traits: Robustness Ability to maintain critical functions in crisis Minimization of direct and indirect Resourcefulness losses from hazards through enhanced Ability to effectively manage crisis as it resistance and robustness to extreme unfolds events, as well as more effective Rapid Recovery recovery strategies. Reconstitute normal operations quickly and effectively Redundancy Backup resources to support originals Per: National Infrastructure Advisory Council, 2009 Hazard Sources & Potential Lunar Habitats Potential Hazard Sources Available Habitat Types Impact (Micrometeorite, Debris) Inflatable Hard Vacuum Membrane Extreme Temperature Rigid-Frame Structure Seismic Activity Hybrid Frame- Low Gravity “Bessel Crater” - https://www.lpi.usra.edu/science/kiefer/Education/SSRG2- Craters/craterstructure.html Membrane Radiation Structure Galactic Cosmic Rays (GCR) Subsurface Solar-Emitted Particles (SEP) Variants Malla, et al. (1995) -
The Calendars of India
The Calendars of India By Vinod K. Mishra, Ph.D. 1 Preface. 4 1. Introduction 5 2. Basic Astronomy behind the Calendars 8 2.1 Different Kinds of Days 8 2.2 Different Kinds of Months 9 2.2.1 Synodic Month 9 2.2.2 Sidereal Month 11 2.2.3 Anomalistic Month 12 2.2.4 Draconic Month 13 2.2.5 Tropical Month 15 2.2.6 Other Lunar Periodicities 15 2.3 Different Kinds of Years 16 2.3.1 Lunar Year 17 2.3.2 Tropical Year 18 2.3.3 Siderial Year 19 2.3.4 Anomalistic Year 19 2.4 Precession of Equinoxes 19 2.5 Nutation 21 2.6 Planetary Motions 22 3. Types of Calendars 22 3.1 Lunar Calendar: Structure 23 3.2 Lunar Calendar: Example 24 3.3 Solar Calendar: Structure 26 3.4 Solar Calendar: Examples 27 3.4.1 Julian Calendar 27 3.4.2 Gregorian Calendar 28 3.4.3 Pre-Islamic Egyptian Calendar 30 3.4.4 Iranian Calendar 31 3.5 Lunisolar calendars: Structure 32 3.5.1 Method of Cycles 32 3.5.2 Improvements over Metonic Cycle 34 3.5.3 A Mathematical Model for Intercalation 34 3.5.3 Intercalation in India 35 3.6 Lunisolar Calendars: Examples 36 3.6.1 Chinese Lunisolar Year 36 3.6.2 Pre-Christian Greek Lunisolar Year 37 3.6.3 Jewish Lunisolar Year 38 3.7 Non-Astronomical Calendars 38 4. Indian Calendars 42 4.1 Traditional (Siderial Solar) 42 4.2 National Reformed (Tropical Solar) 49 4.3 The Nānakshāhī Calendar (Tropical Solar) 51 4.5 Traditional Lunisolar Year 52 4.5 Traditional Lunisolar Year (vaisnava) 58 5. -
Multi-Lunar Day Polar Missions with a Solar-Only Rover. A. Colaprete1, R
Survive the Lunar Night Workshop 2018 (LPI Contrib. No. 2106) 7007.pdf Multi-Lunar Day Polar Missions with a Solar-Only Rover. A. Colaprete1, R. C. Elphic1, M. Shirley1, M. Siegler2, 1NASA Ames Research Center, Moffett Field, CA, 2Planetary Science Institute, Tucson, AZ Introduction: Resource Prospector (RP) was a lu- Polar Solar “Oases”: Numerous studies have nar HEOMD/Advanced Exploration Systems volatiles identified regions near the lunar poles that have sus- prospecting mission developed for potential flight in tained periods of solar illumination. In some places the early 2020s. The mission includes a rover-borne these periods of sustained sunlight extend across sever- payload that (1) can locate surface and near-subsurface al lunations, while others have very short (24-48 hours) volatiles, (2) excavate and analyze samples of the vola- nights. A study was conducted to evaluate if the RP tile-bearing regolith, and (3) demonstrate the form, rover system could take advantage of these “oases” to extractability and usefulness of the materials. The pri- survive the lunar night. While the Earth would set, as mary mission goal for RP is to evaluate the In-Situ seen by the rover, every approximately 2-weeks, these Resource Utilization (ISRU) potential of the lunar “oases” could provide sufficient power, and have lu- poles, to determine their utility within future NASA nar-nights short enough, for the rover system to sur- and commercial spaceflight architectures. While the vive. current RP rover design did not require a system that Results: The study focused in the area surrounding could survive lunar nights, it has been demonstrated the north pole crater Hermite-A (primarily due to the that the current design could conduct multi-lunar day fidelity of existing traverse planner data sets in this missions by taking advantage of areas that receive pro- area). -
Lecture 6: Where Is the Sun?
4.430 Daylighting Massachusetts Institute of Technology Christoph Reinhart Department of Architecture 4.430 Where is the sun? Building Technology Program Goals for This Week Where is the sun? Designing Static Shading Systems MIT 4.430 Daylighting, Instructor C Reinhart 1 1 MISC Meeting on group projects Reduce HDR image size via pfilt –x 800 –y 550 filne_name_large.pic > filename_small>.pic Note: pfilt is a Radiance program. You can find further info on pfilt by googeling: “pfilt Radiance” MIT 4.430 Daylighting, Instructor C Reinhart 2 2 Daylight Factor Hand Calculation Mean Daylight Factor according to Lynes Reinhart & LoVerso, Lighting Research & Technology (2010) Move into the building, design the facade openings, room dimensions and depth of the daylit area. Determine the required glazing area using the Lynes formula. A glazing = required glazing area A total = overall interior surface area (not floor area!) R mean = area-weighted mean surface reflectance vis = visual transmittance of glazing units = sun angle 3 ‘Validation’ of Daylight Factor Formula Reinhart & LoVerso, Lighting Research & Technology (2010) Graph of mean daylighting factor according to Lynes formula v. Radiance removed due to copyright restrictions. Source: Figure 5 in Reinhart, C. F., and V. R. M. LoVerso. "A Rules of Thumb Based Design Sequence for Diffuse Daylight." Lighting Research and Technology 42, no. 1 (2010): 7-32. Comparison to Radiance simulations for 2304 spaces. Quality control for simulations. LEED 2.2 Glazing Factor Formula Graph of mean daylighting factor according to LEED 2.2 v. Radiance removed due to copyright restrictions. Source: Figure 11 in Reinhart, C. F., and V. -
Lunar Sourcebook : a User's Guide to the Moon
3 THE LUNAR ENVIRONMENT David Vaniman, Robert Reedy, Grant Heiken, Gary Olhoeft, and Wendell Mendell 3.1. EARTH AND MOON COMPARED fluctuations, low gravity, and the virtual absence of any atmosphere. Other environmental factors are not The differences between the Earth and Moon so evident. Of these the most important is ionizing appear clearly in comparisons of their physical radiation, and much of this chapter is devoted to the characteristics (Table 3.1). The Moon is indeed an details of solar and cosmic radiation that constantly alien environment. While these differences may bombard the Moon. Of lesser importance, but appear to be of only academic interest, as a measure necessary to evaluate, are the hazards from of the Moon’s “abnormality,” it is important to keep in micrometeoroid bombardment, the nuisance of mind that some of the differences also provide unique electrostatically charged lunar dust, and alien lighting opportunities for using the lunar environment and its conditions without familiar visual clues. To introduce resources in future space exploration. these problems, it is appropriate to begin with a Despite these differences, there are strong bonds human viewpoint—the Apollo astronauts’ impressions between the Earth and Moon. Tidal resonance of environmental factors that govern the sensations of between Earth and Moon locks the Moon’s rotation working on the Moon. with one face (the “nearside”) always toward Earth, the other (the “farside”) always hidden from Earth. 3.2. THE ASTRONAUT EXPERIENCE The lunar farside is therefore totally shielded from the Earth’s electromagnetic noise and is—electro- Working within a self-contained spacesuit is a magnetically at least—probably the quietest location requirement for both survival and personal mobility in our part of the solar system. -
Solar Photovoltaic (PV) Site Assessment
Solar Photovoltaic (PV) Site Assessment Item Type text; Book Authors Franklin, Ed Publisher College of Agriculture, University of Arizona (Tucson, AZ) Download date 29/09/2021 11:10:49 Item License http://creativecommons.org/licenses/by-nc-sa/4.0/ Link to Item http://hdl.handle.net/10150/625447 az1697 August 2017 Solar Photovoltaic (PV) Site Assessment Dr. Ed Franklin Introduction An important consideration when installing a solar photovoltaic (PV) array for residential, commercial, or agricultural operations is determining the suitability of the site. A roof-top location for a residential application may have fewer options due to limited space (roof size), type of roofing material (such as a sloped shingle, or a flat roof), the orientation (south, east, or west), and roof-mounted structures such as vent pipe, chimney, heating & cooling units. A location with open space may utilize a ground-mount system or pole-mount system. Determining the physical location of a solar PV array is a critical step to optimize energy output performance of the system. The ideal location is where solar modules are exposed to full sunshine from sun up to sun down without worry about shade cast on the modules from trees, power poles, guide wires, vent pipes, or nearby buildings, or the changing location of the sun. In the Northern Hemisphere, solar PV arrays are oriented to the south toward the Equator. Over the course of a calendar year, the sun’s altitude (height) in the sky changes. For example, during the month of June, the sun reaches its highest point in the sky on June 21 at 12:00 noon (summer solstice), and the sun Figure 1. -
Workshop Report
WORKSHOP REPORT July 2019 This report was compiled by Andrew Petro, Anna Schonwald, Chris Britt, Renee Weber, Jeff Sheehy, Allison Zuniga, and Lee Mason. Contents Page EXECUTIVE SUMMARY AND RECOMMENDATIONS 2 WORKSHOP SUMMARY 5 Workshop Purpose and Scope 5 Background and Objectives 5 Workshop Agenda 6 Overview of Lunar Day/Night Environmental Conditions 7 Lessons Learned From Missions That Have Survived Lunar Night 8 Science Perspective 9 Exploration Perspective 10 Evolving Requirements from Survival to Continuous Operations for Science, 11 Exploration, and Commercial Activities Power Generation, Storage and Distribution - State of the Art, Potential 13 Solutions, and Technology Gaps Thermal Management Systems, Strategies, and Component Design Features - 16 State of the Art, Potential Solutions, and Technology Gaps The Economic Business Case for Creating Lunar Infrastructure Services and 18 Lunar Markets International Space University Summer Project “Lunar Night Survival” 20 Open Discussion Summary 21 APPENDIX A: Workshop Organizing Committee and Participants 23 APPENDIX B: Poster Session Participants 28 1 Survive and Operate Through the Lunar Night WORKSHOP REPORT June 2019 EXECUTIVE SUMMARY AND RECOMMENDATIONS The lunar day/night cycle, which at most locations on the Moon, includes fourteen Earth days of continuous sunlight followed by fourteen days of continuous darkness and extreme cold presents one of the most demanding environmental challenge that will be faced in the exploration of the solar system. Due to the lack of a moderating atmosphere, temperatures on the lunar surface can range from as high as +120 C during the day to as low as -180 C during the night. Permanently shadowed regions can be even colder. -
Lunar Motion Motion A
2 Lunar V. Lunar Motion Motion A. The Lunar Calendar Dr. Bill Pezzaglia B. Motion of Moon Updated 2012Oct30 C. Eclipses 3 1. Phases of Moon 4 A. The Lunar Calendar 1) Phases of the Moon 2) The Lunar Month 3) Calendars based on Moon b). Elongation Angle 5 b.2 Elongation Angle & Phase 6 Angle between moon and sun (measured eastward along ecliptic) Elongation Phase Configuration 0º New Conjunction 90º 1st Quarter Quadrature 180º Full Opposition 270º 3rd Quarter Quadrature 1 b.3 Elongation Angle & Phase 7 8 c). Aristarchus 275 BC Measures the elongation angle to be 87º when the moon is at first quarter. Using geometry he determines the sun is 19x further away than the moon. [Actually its 400x further !!] 9 Babylonians (3000 BC) note phases are 7 days apart 10 2. The Lunar Month They invent the 7 day “week” Start week on a) The “Week” “moon day” (Monday!) New Moon First Quarter b) Synodic Month (29.5 days) Time 0 Time 1 week c) Spring and Neap Tides Full Moon Third Quarter New Moon Time 2 weeks Time 3 weeks Time 4 weeks 11 b). Stone Circles 12 b). Synodic Month Stone circles often have 29 stones + 1 xtra one Full Moon to Full Moon off to side. Originally there were 30 “sarson The cycle of stone” in the outer ring of Stonehenge the Moon’s phases takes 29.53 days, or ~4 weeks Babylonians measure some months have 29 days (hollow), some have 30 (full). 2 13 c1). Tidal Forces 14 c). Tides This animation illustrates the origin of tidal forces.