DIEL RHYTHMS of BEHAVIOR in JUVENILE PINK SALMON (Oncorhynchus Gorbuscha Walbaum)
Total Page:16
File Type:pdf, Size:1020Kb
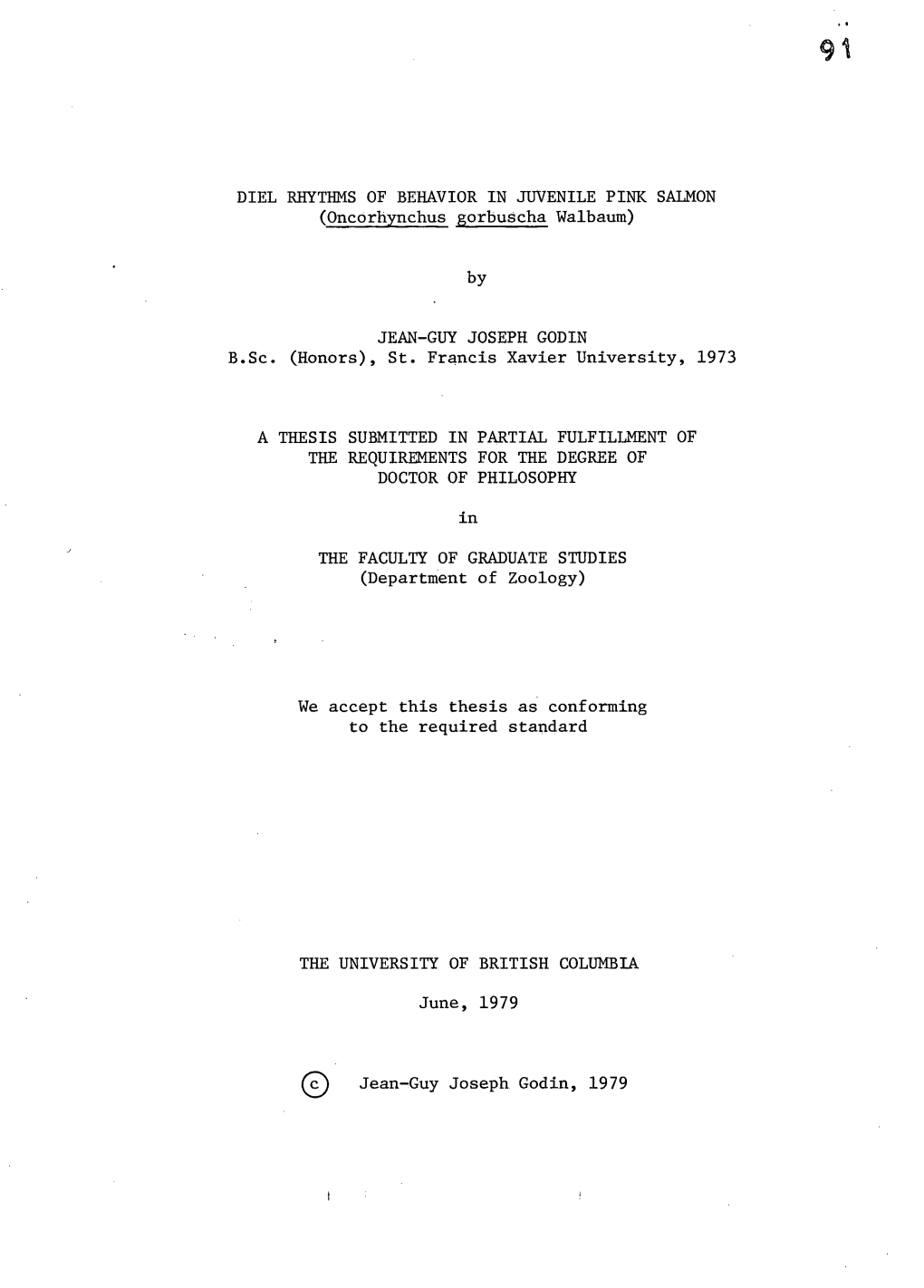
Load more
Recommended publications
-
A Molecular Perspective of Human Circadian Rhythm Disorders Nicolas Cermakian* , Diane B
Brain Research Reviews 42 (2003) 204–220 www.elsevier.com/locate/brainresrev Review A molecular perspective of human circadian rhythm disorders Nicolas Cermakian* , Diane B. Boivin Douglas Hospital Research Center, McGill University, 6875 LaSalle boulevard, Montreal, Quebec H4H 1R3, Canada Accepted 10 March 2003 Abstract A large number of physiological variables display 24-h or circadian rhythms. Genes dedicated to the generation and regulation of physiological circadian rhythms have now been identified in several species, including humans. These clock genes are involved in transcriptional regulatory feedback loops. The mutation of these genes in animals leads to abnormal rhythms or even to arrhythmicity in constant conditions. In this view, and given the similarities between the circadian system of humans and rodents, it is expected that mutations of clock genes in humans may give rise to health problems, in particular sleep and mood disorders. Here we first review the present knowledge of molecular mechanisms underlying circadian rhythmicity, and we then revisit human circadian rhythm syndromes in light of the molecular data. 2003 Elsevier Science B.V. All rights reserved. Theme: Neural basis of behavior Topic: Biological rhythms and sleep Keywords: Circadian rhythm; Clock gene; Sleep disorder; Suprachiasmatic nucleus Contents 1 . Introduction ............................................................................................................................................................................................ 205 -
Circadian Phase Sleep and Mood Disorders (PDF)
129 CIRCADIAN PHASE SLEEP AND MOOD DISORDERS ALFRED J. LEWY CIRCADIAN ANATOMY AND PHYSIOLOGY SCN Efferent Pathways Anatomy Not much is known about how the SCN entrains overt circadian rhythms. We know that the SCN is the master The Suprachiasmatic Nucleus: Locus of the pacemaker, but regarding its regulation of the rest/activity Biological Clock cycle, core body temperature rhythm and cortisol rhythm, Much is known about the neuroanatomic connections of among others, it is not clear if there is a humoral factor or the circadian system. In vertebrates, the locus of the biologi- neural connection that transmits the SCN’s efferent signal; cal clock (the endogenous circadian pacemaker, or ECP) however, a great deal is known about the efferent neural pathway between the SCN and pineal gland. that drives all circadian rhythms is in the hypothalamus, specifically, the suprachiasmatic nucleus (SCN)(1,2).This paired structure derives its name because it lies just above The Pineal Gland the optic chiasm. It contains about 10,000 neurons. The In mammals, the pineal gland is located in the center of molecular mechanisms of the SCN are an active area of the brain; however, it lies outside the blood–brain barrier. research. There is also a great deal of interest in clock genes Postganglionic sympathetic nerves (called the nervi conarii) and clock components of cells in general, not just in the from the superior cervical ganglion innervate the pineal (4). SCN. The journal Science designated clock genes as the The preganglionic neurons originate in the spinal cord, spe- second most important breakthrough for the recent year; cifically in the thoracic intermediolateral column. -
Control Theory Approach to the Study of Circadian Leaf Movement in Trifolium Repens
CONTROL THEORY APPROACH TO THE STUDY OF CIRCADIAN LEAF MOVEMENT IN TRIFOLIUM REPENS by G.R. Robinson B..Sc. Hons.) submitted in fulfilment of the requirements for the degree of Doctor of Philosophy. UNIVERSITY OF TASMANIA HOBART JANUARY, 1979 Thithesis.contains.no material which has been laccepted for the award of any.other.degree . or diploma in any university. To.the best of my knowledge and.belief, the thes is contains.no material previously published . or written by another person except where due reference is made in the text. G.R. Robinson CONTENTS Page SUMMARY ACKNOWLEDGEMENTS iv CHAPTER ONE : GENERAL INTRODUCTION CHAPTER TWO : CIRCADIAN RHYTHMS IN BIOLOGICAL SYSTEMS 1.1 Introduction 5 2.2 Occurrence 6 . 2.3 Characteristics of circadian systems. (a) Light effects 8 (b) Temperature effects . 10 (c) Chemical effects 12 2.4 ModelS:, ,-; -14 CHAPTER THREE : CONTROL THEORY AND ITS APPLICATION ' TO BIOLOGICAL SYSTEMS 5.1 Introduction 18 3.2 Basic concepts 18 3.3 Linear systems . 21 :3.4 The Laplace transformand system transfer .function 23 3.5 System frequency response 25 :3.6 .Relationahip.between frequency response and 25 impulse response 3.7 Determination of frequency.response 27 . (1) Transient inputs 27 (2) Continuous inputs :3.8 Matching a . transfer function to the 28 frequency response Page 3.9 Frequency response for a biological system 31 1.10 Examples of application to biological systems -34 1.11Pulse-phase response curves 36 CHAPTER FOUR : ANALYTICAL METHODS 4.1 Introduction 44 4.2 The discrete Fourier transform 45 4.3 Spectral analysis from a least squares viewpoint 47 4.3.1 Estimating amplitude and phase 48 4.3.2 Estimation of frequency 50 4.3.3 Several periodic terms 51 4.4 Properties of the DFT 51 4.4.1 Leakage 52 4.4.2 Aliasing 58 4.5 Reliability of spectral estimates 64 4.5.1 Presence of frequency components 64 4.5.2 Reliability of frequency, amplitude and 66 phase estimates 4.5.3 Combination of estimates 69 4.6 Oscillations with nonstationary parameters 71 4.7 Future developments 72 CHAPTER FIVE : EXPERIMENTAL METHOD . -
Biological Rhythms Workshop I: Introduction to Chronobiology
Downloaded from symposium.cshlp.org on November 30, 2008 - Published by Cold Spring Harbor Laboratory Press Biological Rhythms Workshop I: Introduction to Chronobiology S. J. Kuhlman, S. R. Mackey and J. F. Duffy Cold Spring Harb Symp Quant Biol 2007 72: 1-6 Access the most recent version at doi:10.1101/sqb.2007.72.059 References This article cites 24 articles, 6 of which can be accessed free at: http://symposium.cshlp.org/content/72/1.refs.html Article cited in: http://symposium.cshlp.org/content/72/1.abstract.html#related-urls Email alerting Receive free email alerts when new articles cite this article - sign up in the box at the service top right corner of the article or click here To subscribe to Cold Spring Harbor Symposia on Quantitative Biology go to: http://symposium.cshlp.org/subscriptions/ © Cold Spring Harbor Laboratory Press Downloaded from symposium.cshlp.org on November 30, 2008 - Published by Cold Spring Harbor Laboratory Press Biological Rhythms Workshop I: Introduction to Chronobiology S.J. KUHLMAN,* S.R. MACKEY,†‡ AND J.F. DUFFY§ *Cold Spring Harbor Laboratory, Cold Spring Harbor, New York 11724; †Department of Biology, The Center for Research on Biological Clocks, Texas A&M University, College Station, Texas 77843-3258; §Division of Sleep Medicine, Harvard Medical School and Brigham and Women’s Hospital, Boston, Massachusetts 02115 In this chapter, we present a series of four articles derived from a Introductory Workshop on Biological Rhythms presented at the 72nd Annual Cold Spring Harbor Symposium on Quantitative Biology: Clocks and Rhythms. A diverse range of species, from cyanobacteria to humans, evolved endogenous biological clocks that allow for the anticipation of daily variations in light and temperature. -
Circadian Rhythm Phase Shifts Caused by Timed Exercise Vary with Chronotype in Young Adults
University of Kentucky UKnowledge Theses and Dissertations--Kinesiology and Health Promotion Kinesiology and Health Promotion 2019 CIRCADIAN RHYTHM PHASE SHIFTS CAUSED BY TIMED EXERCISE VARY WITH CHRONOTYPE IN YOUNG ADULTS J. Matthew Thomas University of Kentucky, [email protected] Digital Object Identifier: https://doi.org/10.13023/etd.2019.406 Right click to open a feedback form in a new tab to let us know how this document benefits ou.y Recommended Citation Thomas, J. Matthew, "CIRCADIAN RHYTHM PHASE SHIFTS CAUSED BY TIMED EXERCISE VARY WITH CHRONOTYPE IN YOUNG ADULTS" (2019). Theses and Dissertations--Kinesiology and Health Promotion. 64. https://uknowledge.uky.edu/khp_etds/64 This Doctoral Dissertation is brought to you for free and open access by the Kinesiology and Health Promotion at UKnowledge. It has been accepted for inclusion in Theses and Dissertations--Kinesiology and Health Promotion by an authorized administrator of UKnowledge. For more information, please contact [email protected]. STUDENT AGREEMENT: I represent that my thesis or dissertation and abstract are my original work. Proper attribution has been given to all outside sources. I understand that I am solely responsible for obtaining any needed copyright permissions. I have obtained needed written permission statement(s) from the owner(s) of each third-party copyrighted matter to be included in my work, allowing electronic distribution (if such use is not permitted by the fair use doctrine) which will be submitted to UKnowledge as Additional File. I hereby grant to The University of Kentucky and its agents the irrevocable, non-exclusive, and royalty-free license to archive and make accessible my work in whole or in part in all forms of media, now or hereafter known. -
White Tank Mountain Regional Park
White Tank Mountain Regional Park Master Plan Update 2014-2034 {This page intentionally left blank.} {This page intentionally left blank.} {This page intentionally left blank.} Acknowledgements This master plan update was a collaborative process that involved the guidance and expertise of many. The Maricopa County Parks and Recreation Department would like to thank the Planning Team who committed their time to monthly meetings and document review; likewise to the Stakeholder Advisory Group who took time out of their personal lives to provide their invaluable input. Planning Team Stakeholder Advisory Group R.J. Cardin, Director Jamil Coury Jennifer Waller, Operations Manager Linda Gilgosch Jennifer Johnston, West Side Superintendent John Pesock Raymond Schell, Park Supervisor Frank Salowitz Michele Kogl, Planning and Development Manager Allen Ockenfels, Trails Development Manager Fareed Abou-Haidar, GIS Technician Leigh Johnson, Park Planner This Master Plan update was also made possible by the contributions and guidance of the following: • Maricopa County Board of Supervisors o Denny Barney, District 1 o Steve Chucri, District 2 o Andy Kunasek, District 3 o Clint L. Hickman, District 4 o Mary Rose Wilcox, District 5 • Maricopa County Parks and Recreation Commission o Jack Stapley, District 2 o Anne Lynch, District 3 o Dr. Robert Branch, District 4 o Carlton Yoshioka , Member-at-Large o Rod Jarvis, Member-at-Large • Maricopa County Parks and Recreation Department staff • Maricopa County Sheriff’s Office, Mountain Patrol Division • Bill “Doc” Talboys, Interpretive Ranger, MCPRD (retired) • Shelly Rasmussen, Interpretive Ranger, MCPRD (retired) • Public meeting participants The Department would also like to thank its agency partners at the City of Surprise, City of Buckeye, Arizona Game and Fish Department, and the Bureau of Land Management - Phoenix District Office for their input and guidance. -
Chapter 1 Photosynthesis
Revised Edition: 2016 ISBN 978-1-283-50684-7 © All rights reserved. Published by: The English Press 48 West 48 Street, Suite 1116, New York, NY 10036, United States Email: [email protected] Table of Contents Chapter 1 - Photosynthesis Chapter 2 - Photomorphogenesis Chapter 3 - Visual System Chapter 4 - Circadian Rhythm Chapter 5 - Bioluminescence Chapter 6 - Ultraviolet Chapter 7 - Light Therapy Chapter 8 - Light Effects on Circadian Rhythm and Scotobiology WT ________________________WORLD TECHNOLOGIES________________________ Chapter 1 Photosynthesis WT Composite image showing the global distribution of photosynthesis, including both ocea- nic phytoplankton and vegetation Overall equation for the type of photosynthesis that occurs in plants Photosynthesis is a process that converts carbon dioxide into organic compounds, es- pecially sugars, using the energy from sunlight. Photosynthesis occurs in plants, algae, ________________________WORLD TECHNOLOGIES________________________ and many species of bacteria, but not in archaea. Photosynthetic organisms are called photoautotrophs, since they can create their own food. In plants, algae, and cyanoba- cteria, photosynthesis uses carbon dioxide and water, releasing oxygen as a waste product. Photosynthesis is vital for all aerobic life on Earth. As well as maintaining the normal level of oxygen in the atmosphere, nearly all life either depends on it directly as a source of energy, or indirectly as the ultimate source of the energy in their food (the exceptions are chemoautotrophs that live in rocks or around deep sea hydrothermal vents). The rate of energy capture by photosynthesis is immense, approximately 100 terawatts, which is about six times larger than the power consumption of human civilization. As well as energy, photosynthesis is also the source of the carbon in all the organic compounds within organisms' bodies. -
Cycles of Nature. an Introduction to Biological Rhythms. INSTITUTION National Science Teachers Association, Washington, D.C
;' DOCUMENT RESUME ED 328 446 SE 051 926 AUTHOR Ahlgren, Andrew; Halberg, Franz TITLE Cycles of Nature. An Introduction to Biological Rhythms. INSTITUTION National Science Teachers Association, Washington, D.C. REPORT NO ISBN-0-87355-089-7 PUB DATE 90 NOTE 96p. AVAILABLE FROMNational Science Teachers Association, 1742 Connecticut Avenue, Washington, DC 20009 ($12.00). PUB TYPE Guides - Classroom Use - Guides (For Teachers) (052) EDRS PRICE MF01 Plus Postage. PC Not Available from EDRS. DESCRIPTORS Biological Influences; *Biology; Graphs; Human Body; Laboratory Procedures; Medicine; *Science Activities; *Science Curriculum; Science Education; Scientific Concepts; Secondary Education; *Secondary School Science IDENTIFIERS *Chronobiology ABSTRACT This book is an outlined for the short study (1- to 2-weeks) of chronobiology, a field of science that explores the relationships between time and biological functions. It develops step-by-step the reasoning that leads to the current scientific understanding of biological rhythms. The unit can be inserted into a standard middle or high school biology course. Because the scientific study of biological rhythms begins with data, Chapter 1 provides a brief review of the ways to collect, graph, and interpret data. Chapter 2 introduces some of the cycles in nature, especially those of the human body-from dream cycles to menstruation to body temperature. Chapter 3 explores how these cycles come about and explains the differences between external and internal influences. Chapter 4 explores the internal uorkings of organisms to determine whether there is a single master source of timing information that synchronizes an organism's many interacting cycles. Chapter 5 discusses the impact of rhythms on society and asks how an understanding of them could bring progress in medicine, work schedules, and everyday life. -
Are There Circadian Clocks in Non-Photosynthetic Bacteria?
biology Review Are There Circadian Clocks in Non-Photosynthetic Bacteria? Francesca Sartor 1, Zheng Eelderink-Chen 1, Ben Aronson 2, Jasper Bosman 3, Lauren E. Hibbert 4, Antony N. Dodd 4,*, Ákos T. Kovács 5,* and Martha Merrow 1,* 1 Institute of Medical Psychology, Medical Faculty, LMU Munich, 80336 Munich, Germany; [email protected] (F.S.); [email protected] (Z.E.-C.) 2 Department of Biology, University of Redlands, Redlands, CA 92373, USA; [email protected] 3 Bioinformatics, Hanzehogeschool Groningen, 9747 AS Groningen, The Netherlands; [email protected] 4 School of Biological Sciences, University of Bristol, Bristol BS8 1TQ, UK; [email protected] 5 Bacterial Interactions and Evolution Group, Department of Biotechnology and Biomedicine, Technical University of Denmark, 2800 Kgs. Lyngby, Denmark * Correspondence: [email protected] (A.N.D.); [email protected] (Á.T.K.); [email protected] (M.M.); Tel.: +44-117-394-1176 (A.N.D.); +45-4525-2527 (A.T.K.); +49-89-218075-650 (M.M.) Received: 6 March 2019; Accepted: 15 May 2019; Published: 22 May 2019 Abstract: Circadian clocks in plants, animals, fungi, and in photosynthetic bacteria have been well-described. Observations of circadian rhythms in non-photosynthetic Eubacteria have been sporadic, and the molecular basis for these potential rhythms remains unclear. Here, we present the published experimental and bioinformatical evidence for circadian rhythms in these non-photosynthetic Eubacteria. From this, we suggest that the timekeeping functions of these organisms will be best observed and studied in their appropriate complex environments. Given the rich temporal changes that exist in these environments, it is proposed that microorganisms both adapt to and contribute to these daily dynamics through the process of temporal mutualism. -
SLTBR Board of Directors: 2012-2013
Society for Light Treatment and Biological Rhythms Program and Abstracts: Volume 25 25th Annual Meeting June 21-23, 2013 Starling Hotel & Convention Center Geneva, Switzerland www.sltbr2013.org Matthäus Willeit, SLTBR President 2012-2014 Scientific Committee: Matthäus Willeit (chair), Anna Wirz-Justice, Namni Goel, and Farhad Hafezi Local arrangements are made courtesy of: Center for Environmental Therapeutics - Europe & The University of Geneva Citation Format: Society for Light Treatment and Biol Rhythms Abst 2013; 25:xx. Contact SLTBR at [email protected] www.sltbr.org © 2013 Society for Light Treatment and Biological Rhythms. All rights reserved. 2 MESSAGE FROM OUR LOCAL HOST Dear Friends and Colleagues, We are delighted to welcome you again to Geneva! As the local organizing host and together with the program committee members, we look forward to an exciting program that combines multiple disciplines and research topics. I believe that the roundtable will provide interesting discussions about the latest trends and findings in the field. And, I would like to welcome and thank our first-time sponsors to the meeting – Carex Health Brands (USA), Nature Bright (USA), Von Hoff AG, Valkee Oy, and Sanalux GmbH (Switzerland). I would also like to thank our returning sponsors for their continued support of SLTBR – Phillips (The Netherlands), Lumie (UK), Medi-Lum SàrL (Switzerland), Northern Lights (Canada), and Phytolis AG (Switzerland). I wish all of you a very productive and enlightening congress and unforgettable moments in Geneva. Sincerely, Farhad Hafezi, MD PhD Professor and Chair of Ophthalmology University of Geneva 3 SLTBR recognizes and thanks the following companies for their contribution and support of the 25th Annual SLTBR Meeting: Corporate Gold Sponsors Corporate Silver Sponsors 4 Speaker Sponsors Academic Sponsor/Local Host 5 SLTBR Board of Directors: 2012-2013 President Student Director Matthäus Willeit, MD, John Hanifin Dept. -
Circadian Rhythm and Their Significance in Relation To
Journal of Entomology and Zoology Studies 2018; 6(4): 1861-1866 E-ISSN: 2320-7078 P-ISSN: 2349-6800 Circadian rhythm and their significance in JEZS 2018; 6(4): 1861-1866 © 2018 JEZS relation to physiological functions of animals: A Received: 24-05-2018 Accepted: 25-06-2018 review Sohan Vir Singh Animal Physiology Division ICAR-National Dairy Research Sohan Vir Singh and Sunil Kumar Institute, Karnal, Haryana, India Abstract Circadian rhythm regulates the physiological processes of animals. The ambient conditions viz. Sunil Kumar Animal Physiology Division wavelength, intensity of light, timing and duration of the light stimulus, temperature etc deregulates the ICAR-National Dairy Research circadian rhythm. Circadian rhythms are generated at the cellular level in living organisms. Sleep–wake Institute, Karnal, Haryana, cycle, fattening, hibernation, reproductive behavior and migration pattern/ behavior in birds are India controlled by biological rhythms in animals. Biological rhythms are controlled endogenously by self- contained circadian clocks. The timing of sleep and wakefulness under natural conditions is in synchrony with the circadian control of the sleep cycle and all other circadian-controlled rhythms. Generally, the circadian rhythm is regulated by the light/dark cycle of the solar day, but it also persists during constant conditions. Change in the photoperiod (daylength) is the most important environmental factor for the deviation in physiology and behavior, timing of migration, hibernation, reproduction etc. Arctic animals (ptarmigan, reindeer) show circadian rhythms only in the parts of the year that have daily sunrises and sunsets. Circadian clocks have been reported in each and every mammalian cell including cardiovascular system, such as cardiomyocytes and vascular smooth muscle cells. -
Circadian Rhythms Contents Page GENERAL PROPERTIES of CIRCADIAN RHYTHMS
Chapter 3 Circadian Rhythms Contents Page GENERAL PROPERTIES OF CIRCADIAN RHYTHMS . 37 THE CELLULAR CLOCK . .. 38 THE PACEMAKER IN THE BRAIN . ...........40 HUMAN CIRCADIAN RHYTHMS . 41 The Body Circadian . 42 The Timing of Sleep . .. 45 Human Performance . 47 DISRUPTION OF CIRCADIAN RHYTHMS . ,...49 Aging and the Body Clock . .. 49 Sleep Disorders . 51 Chronobiology and Mood Disorders . .. 51 CONTROLLING CIRCADIAN RHYTHMS IN HUMANS . 53 Light . .. 53 Melatonin . 55 Benzodiazepines . .. 56 Other Chemical Substances and Diet . 57 Activity . ,. 57 SUMMARY AND CONCLUSIONS . .+....*. 58 CHAPTER PREFERENCES . 58 Boxes Box Page 3-A. Circadian Rhythms and Drugs . ● . 43 3-B. Cycles That Last From Minutes To Days . .44 3-C. Napping . 46 3-D. Jet Lag . 48 Figures Figure Page 3-1. Circadian Rhythm . 37 3-2. Synchronized and Free-Running Circadian Rhythms . 38 3-3. Phase Response Curve. -. 39 3-4. Gene Expression in the Pacemaker . 40 3-5. The Transplanted Pacemaker . ....*.,. 41 3-6. Human Circadian Rhythms . .42 3-7. Human Circadian Rhythms in the Absence of Time Cues . 46 3-8. Sleepiness During the Day . .47 3-9. Circadian Rhythms of Alertness . 49 3-10. Aging and the Pacemaker . .*.....* . 50 3-11. Levels of Light . 52 3-12. Resetting the Human Pacemaker With Light. ....*.., . .54 3-13. Bright Light and Air Travel . ....,.*, . 55 3-14. Melatonin Rhythms and Light . .. 56 Table Table Page 3-1. Differences Between Rapid Eye Movement (REM) Sleep and Slow Wave Sleep (SWS) . 45 Chapter 3 Circadian Rhythms Many biological functions wax and wane in An entraining agent can actually reset, or cycles that repeat each day, month, or year.