Evolution of Digestive Enzymes and Dietary Diversification in Birds
Total Page:16
File Type:pdf, Size:1020Kb
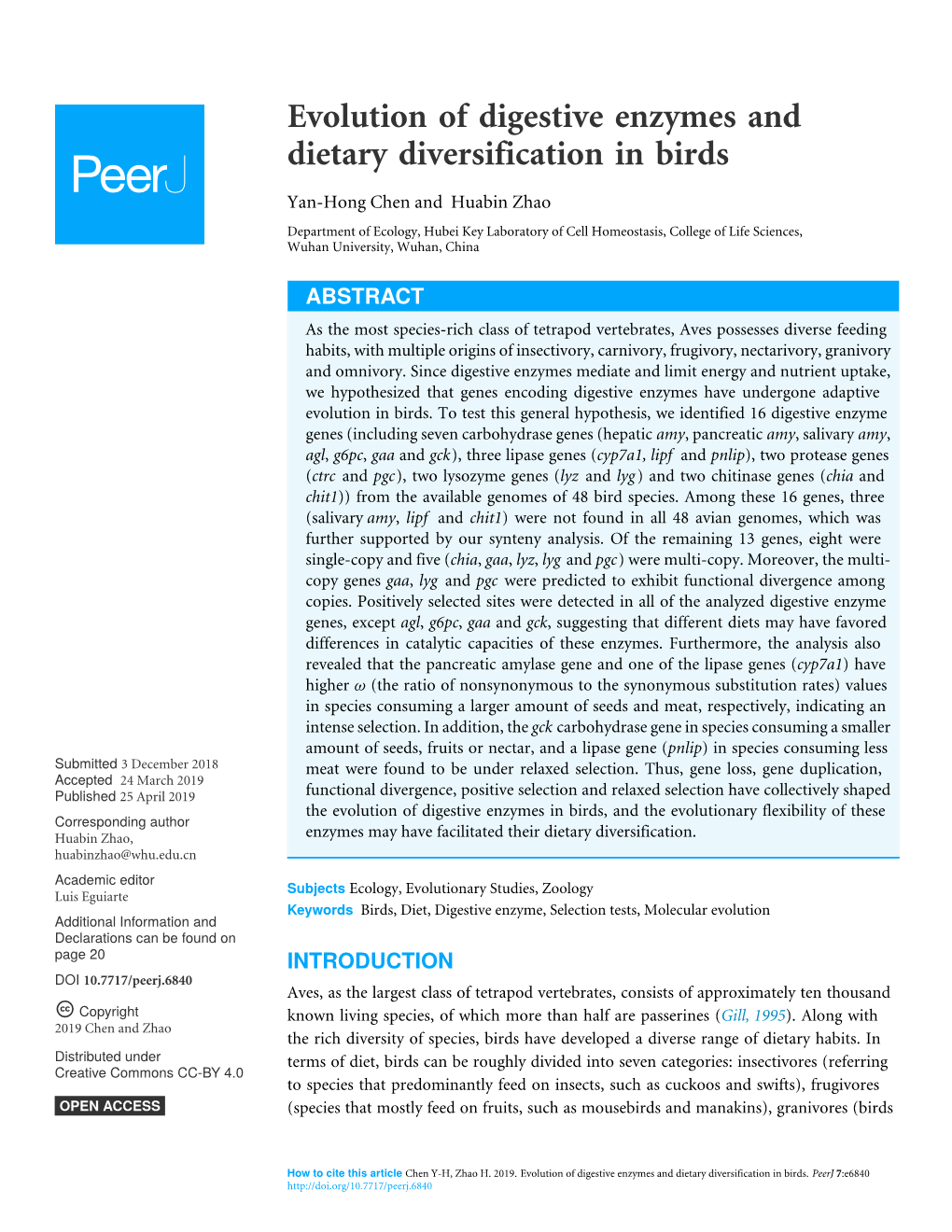
Load more
Recommended publications
-
Analyse Structurale Et Fonctionnelle De La Préséniline-1 Humaine : Implications Dans La Maladie D'alzheimer
SÉBASTIEN HÉBERT ANALYSE STRUCTURALE ET FONCTIONNELLE DE LA PRÉSÉNILINE-1 HUMAINE : IMPLICATIONS DANS LA MALADIE D’ALZHEIMER Thèse présentée à la Faculté des études supérieures de l’Université Laval dans le cadre du programme de biologie cellulaire et moléculaire pour l’obtention du grade de Philosophiae Doctor (Ph.D.) FACULTÉ DE MÉDECINE UNIVERSITÉ LAVAL QUÉBEC DÉCEMBRE 2003 © Sébastien S. Hébert, 2003 i RÉSUME COURT La préséniline-1 (PS1) mutée est associée à un développement très précoce de la maladie (chez les individus de 24-55 ans) d’Alzheimer. Dans la cellule, la PS1 existe principalement sous forme de fragments (N-terminaux et C-terminaux, respectivement NTFs et CTFs) qui semblent nécessaires à sa fonction et à l’activité γ- secrétase étroitement liée à la formation des peptides β-amyloïdes neurotoxiques. Nous avons étudié l’organisation structurale et fonctionnelle de la PS1, ses fragments, et leurs relations avec les protéines impliquées dans la production des peptides β- amyloïdes. Dans un premier temps, des expériences en levure, confirmées avec des techniques d’immunobuvardage et d’immunoprécipitation en cellules de mammifères, nous ont permis de mettre en évidence un rôle de l’holoprotéine de la PS1 dans la génération des fragments NTFs et CTFs. De plus, il a été possible de démontrer une interaction directe entre PS1 et BACE (enzyme de type aspartyl protéase aussi appelée β-secrétase), les deux joueurs clés dans la production des peptides β- amyloïdes. Finalement, l’étude du rôle fonctionnel de cette interaction nous a fournis des résultats permettant de suggérer une régulation de la PS1 par BACE. -
2006.01) Kr, Kw, Kz, La, Lc, Lk, Lr, Ls, Lu, Ly, Ma, Md, Me, (21
( (51) International Patent Classification: DZ, EC, EE, EG, ES, FI, GB, GD, GE, GH, GM, GT, HN, A61K 48/00 (2006.01) HR, HU, ID, IL, IN, IR, IS, JO, JP, KE, KG, KH, KN, KP, KR, KW, KZ, LA, LC, LK, LR, LS, LU, LY, MA, MD, ME, (21) International Application Number: MG, MK, MN, MW, MX, MY, MZ, NA, NG, NI, NO, NZ, PCT/US20 19/06 1701 OM, PA, PE, PG, PH, PL, PT, QA, RO, RS, RU, RW, SA, (22) International Filing Date: SC, SD, SE, SG, SK, SL, SM, ST, SV, SY, TH, TJ, TM, TN, 15 November 2019 (15. 11.2019) TR, TT, TZ, UA, UG, US, UZ, VC, VN, ZA, ZM, ZW. (25) Filing Language: English (84) Designated States (unless otherwise indicated, for every kind of regional protection available) . ARIPO (BW, GH, (26) Publication Language: English GM, KE, LR, LS, MW, MZ, NA, RW, SD, SL, ST, SZ, TZ, (30) Priority Data: UG, ZM, ZW), Eurasian (AM, AZ, BY, KG, KZ, RU, TJ, 62/768,645 16 November 2018 (16. 11.2018) US TM), European (AL, AT, BE, BG, CH, CY, CZ, DE, DK, 62/769,697 20 November 2018 (20. 11.2018) US EE, ES, FI, FR, GB, GR, HR, HU, IE, IS, IT, LT, LU, LV, 62/778,706 12 December 2018 (12. 12.2018) US MC, MK, MT, NL, NO, PL, PT, RO, RS, SE, SI, SK, SM, TR), OAPI (BF, BJ, CF, CG, Cl, CM, GA, GN, GQ, GW, (71) Applicant: ASKLEPIOS BIOPHARMACEUTICAL, KM, ML, MR, NE, SN, TD, TG). -
DUAL ROLE of CATHEPSIN D: LIGAND and PROTEASE Martin Fuseka, Václav Větvičkab
Biomed. Papers 149(1), 43–50 (2005) 43 © M. Fusek, V. Větvička DUAL ROLE OF CATHEPSIN D: LIGAND AND PROTEASE Martin Fuseka, Václav Větvičkab* a Institute of Organic Chemistry and Biochemistry, CAS, Prague, Czech Republic, and b University of Louisville, Department of Pathology, Louisville, KY40292, USA, e-mail: [email protected] Received: April 15, 2005; Accepted (with revisions): June 20, 2005 Key words: Cathepsin D/Procathepsin D/Cancer/Activation peptide/Mitogenic activity/Proliferation Cathepsin D is peptidase belonging to the family of aspartic peptidases. Its mostly described function is intracel- lular catabolism in lysosomal compartments, other physiological effect include hormone and antigen processing. For almost two decades, there have been an increasing number of data describing additional roles imparted by cathepsin D and its pro-enzyme, resulting in cathepsin D being a specific biomarker of some diseases. These roles in pathological conditions, namely elevated levels in certain tumor tissues, seem to be connected to another, yet not fully understood functionality. However, despite numerous studies, the mechanisms of cathepsin D and its precursor’s actions are still not completely understood. From results discussed in this article it might be concluded that cathepsin D in its zymogen status has additional function, which is rather dependent on a “ligand-like” function then on proteolytic activity. CATHEPSIN D – MEMBER PRIMARY, SECONDARY AND TERTIARY OF ASPARTIC PEPTIDASES FAMILY STRUCTURES OF ASPARTIC PEPTIDASES Major function of cathepsin D is the digestion of There is a high degree of sequence similarity among proteins and peptides within the acidic compartment eukaryotic members of the family of aspartic peptidases, of lysosome1. -
A Novel Thermostable Aspartic Protease from Talaromyces Leycettanus and Its Specific
bioRxiv preprint doi: https://doi.org/10.1101/528265; this version posted January 23, 2019. The copyright holder for this preprint (which was not certified by peer review) is the author/funder. All rights reserved. No reuse allowed without permission. 1 1 RESEARCH ARTICLE 2 3 A novel thermostable aspartic protease from Talaromyces leycettanus and its specific 4 autocatalytic activation through an intermediate transition state 5 6 Yujie Guo1, Tao Tu1, Yaxin Ren1, Yaru Wang1, Yingguo Bai1, Xiaoyun Su1, Yuan 7 Wang1, Bin Yao1, Huoqing Huang1*, Huiying Luo1* 8 9 1Key Laboratory for Feed Biotechnology of the Ministry of Agriculture, Feed Research 10 Institute, Chinese Academy of Agricultural Sciences, Beijing 100081, P. R. China 11 Running title: Insights into a specific auto-activation of proTlAPA1 12 * Corresponding authors. 13 E-mail addresses: [email protected] (H. Luo), [email protected] (H. Huang). 2 bioRxiv preprint doi: https://doi.org/10.1101/528265; this version posted January 23, 2019. The copyright holder for this preprint (which was not certified by peer review) is the author/funder. All rights reserved. No reuse allowed without permission. 14 ABSTRACT 15 Aspartic proteases exhibit optimum enzyme activity under acidic condition and 16 have been extensively used in food, fermentation and leather industries. In this study, 17 a novel aspartic protease precursor (proTlAPA1) from Talaromyces leycettanus was 18 identified and successfully expressed in Pichia pastoris. Subsequently, the 19 auto-activation processing of the zymogen proTlAPA1 was studied by SDS-PAGE 20 and N-terminal sequencing, under different processing conditions. TlAPA1 shared the 21 highest identity of 70.3 % with the aspartic endopeptidase from Byssochlamys 22 spectabilis (GAD91729) and was classified into a new subgroup of the aspartic 23 protease A1 family, based on evolutionary analysis. -
Multiple Functions of Pro-Parts of Aspartic Proteinase Zymogens
View metadata, citation and similar papers at core.ac.uk brought to you by CORE provided by Elsevier - Publisher Connector FEBS Letters 343 (1994) 6-10 ELSEVIER FEBS 13889 Minireview Multiple functions of pro-parts of aspartic proteinase zymogens Gerald Koelsch”, Michael MareSb, Peter Metcalf”, Martin FusekaT* ~~k~ahornaMedical Research ~~~n~t~on and Un~Qer~ityof Oklahoma Hearth Sciences Center, 825 N.E. 13th Street, Oklahoma City, OK 73f04, USA ‘institute of Organic Chem~try and Ei~c~~rn~~r~,Czech Academy of Sciences, Prague, CZ 16~~~~Czech Republic “European Molecular Bioiogy Laboratory, Heidelberg, D-6900, Germany Received 4 March 1994 Abstract The importance of aspartic proteinases in human pathophysiology continues to initiate extensive research. With burgeoning information on their biological functions and structures, the traditional view of the role of activation peptides of aspartic proteinases solely as inhibitors of the active site is changing. These peptide segments, or pro-parts, am deemed important for correct folding, targeting, and control of the activation of aspartic proteinase zymogens. Consequently, the primary structures of pro-parts reflect these functions. We discuss guidelines for formation of hypotheses derived from comparing the physiological function of aspartic proteinases and sequences of their pro-parts. Key words: Aspartic proteinase; Chaperon; Folding; Pro-part; Protein structure; Targeting 1. Introduction teinases starts with the removal of their signal peptides during the passage into the endoplasmic reticulum. The Aspartic proteinases (EC 3.4.23) share a high degree complete activation of eucaryotic zymogens of aspartic of similarity which involves primary structures, extend- proteinases is accomplished by a proteolytic removal of ing through almost identical secondary structural motifs the N-terminal pro-part which is usually 44-50 amino and manifesting a typical bilobal molecular shape [1,2]. -
Demonstration of Pepsinogen C in Human Pancreatic Islets
Gut: first published as 10.1136/gut.28.10.1208 on 1 October 1987. Downloaded from Gllt, 1987, 28, 1208- 1214 Alimentary tract and pancreas Demonstration of pepsinogen C in human pancreatic islets P B SZECSI, LI HALGREEN, SS POULSEN, C K AXELSSON, M DAMKJ/ER-NIELSEN, T KJ/ER, AND B FOLTMANN From The Department of Clinical Chemistry, Hvidovre Hospital; Institute of Pathology, Bispebjerg Hospital; Institute of Biochemical Genzetics, In.stituite of Medical Anatomy, Departnzent B and Department of Clinical Physiology, Glostrup Hospital, University of Copenhagen. Department K of Surgery, Vejle Hospital and Department L ofSurgery, Arhu.s Municipal Hospital, University ofArhus, Denmark. SUMMARY Pancreatic tissue from 16 post mortem kidney donors have been examined for the content of pepsinogens. A zymogen with electrophoretic mobility, isoelectric point and molecular weight equal to that of pepsinogen C of gastric origin was found in all specimens. A comparison between pepsinogen C extracted from pancreatic tissue and gastric mucosa demonstrated immunological identity. Quantitative measurements with a radioimmunoassay showed pepsinogen C concentrations in pancreatic tissue three to 80 times higher than those of blood serum. Immunohistochemical staining gave positive reaction for pepsinogen C only in the alpha cells of the pancreatic islets. http://gut.bmj.com/ Gastric proteases belong to the superfamily of superfamily of aspartic proteases localised outside aspartic proteases (EC 3.4.23). The predominant the gastrointestinal tract have traditionally been gastric proteases of adult mammals are designated designated cathepsin D or E.'" During a series of pepsin A (EC 3.4.23.1) and pepsin C (EC 3.4.23.3). -
Chitin Digestibility Is Dependent on Feeding Behaviors, Which Determine Acidic Chitinase Mrna Levels in Mammalian and Poultry St
www.nature.com/scientificreports OPEN Chitin digestibility is dependent on feeding behaviors, which determine acidic chitinase mRNA Received: 20 October 2017 Accepted: 10 January 2018 levels in mammalian and poultry Published: xx xx xxxx stomachs Eri Tabata1, Akinori Kashimura1, Azusa Kikuchi1, Hiromasa Masuda1, Ryo Miyahara1, Yusuke Hiruma1, Satoshi Wakita1, Misa Ohno1, Masayoshi Sakaguchi1, Yasusato Sugahara1, Vaclav Matoska2, Peter O. Bauer2,3 & Fumitaka Oyama 1 Chitin, a polymer of N-acetyl-D-glucosamine (GlcNAc), functions as a major structural component in chitin-containing organism including crustaceans, insects and fungi. Recently, we reported that acidic chitinase (Chia) is highly expressed in mouse, chicken and pig stomach tissues and that it can digest chitin in the respective gastrointestinal tracts (GIT). In this study, we focus on major livestock and domestic animals and show that the levels of Chia mRNA in their stomach tissues are governed by the feeding behavior. Chia mRNA levels were signifcantly lower in the bovine (herbivores) and dog (carnivores) stomach than those in mouse, pig and chicken (omnivores). Consistent with the mRNA levels, Chia protein was very low in bovine stomach. In addition, the chitinolytic activity of E. coli-expressed bovine and dog Chia enzymes were moderately but signifcantly lower compared with those of the omnivorous Chia enzymes. Recombinant bovine and dog Chia enzymes can degrade chitin substrates under the artifcial GIT conditions. Furthermore, genomes of some herbivorous animals such as rabbit and guinea pig do not contain functional Chia genes. These results indicate that feeding behavior afects Chia expression levels as well as chitinolytic activity of the enzyme, and determines chitin digestibility in the particular animals. -
Molecular and Evolutionary Basis for Survival, Its Failure, And
bioRxiv preprint doi: https://doi.org/10.1101/2021.03.12.435207; this version posted March 13, 2021. The copyright holder for this preprint (which was not certified by peer review) is the author/funder, who has granted bioRxiv a license to display the preprint in perpetuity. It is made available under aCC-BY-NC-ND 4.0 International license. 1 Good host - bad host: molecular and evolutionary basis for survival, its failure, and virulence factors 2 of the zoonotic nematode Anisakis pegreffii 3 4 5 6 7 Željka Trumbić1, Jerko Hrabar2, Nikola Palevich3, Vincenzo Carbone3, Ivona Mladineo4* 8 9 1University Department of Marine Studies, University of Split, 21000 Split, Croatia 10 2Laboratory of Aquaculture, Institute of Oceanography & Fisheries, 21000 Split, Croatia 11 3AgResearch Limited, Grasslands Research Centre, Palmerston North, 4410, New Zealand 12 4Laboratory of Functional Helminthology, Institute of Parasitology, Biology Centre of Czech Academy of 13 Science, 37005 Ceske Budejovice, Czech Republic 14 15 16 17 corresponding author: [email protected] 18 19 20 21 22 23 24 25 26 27 28 29 30 31 32 33 34 35 36 37 38 39 40 41 42 43 44 45 46 47 48 bioRxiv preprint doi: https://doi.org/10.1101/2021.03.12.435207; this version posted March 13, 2021. The copyright holder for this preprint (which was not certified by peer review) is the author/funder, who has granted bioRxiv a license to display the preprint in perpetuity. It is made available under aCC-BY-NC-ND 4.0 International license. 49 Abstract 50 Parasitism is a highly successful life strategy and a driving force in genetic diversity that has evolved many 51 times over. -
Molecular Cloning and the Nucleotide Sequence of Cdna for Embryonic Chicken Pepsinogen: Phylogenetic Relationship with Prochymosin1
J. Biochem. 103, 290-296 (1988) Molecular Cloning and the Nucleotide Sequence of cDNA for Embryonic Chicken Pepsinogen: Phylogenetic Relationship with Prochymosin1 Kensuke Hayashi, * Kiyokazu Agata, * * Makoto Mochii, * * Sadao Yasugi, * Goro Eguehi, and Takeo Mizuno* *Zoological Institute , Faculty of Science, The University of Tokyo, Bunkyo-ku, Tokyo 113; and **Department of Developmental Biology, National Institute for Basic Biology, Okazaki, Aichi 444 Received for publication, September 17, 1987 Embryonic chicken pepsinogen is an aspartyl proteinase that is specifically secreted during the embryonic period in the chicken proventriculus (glandular stomach). To learn the phylogeny of this pepsinogen, we isolated a eDNA clone by screening a Agtll library of embryonic proventricular cDNAs with an antiserum to the embryonic chicken pepsinogen. We obtained a 200-base pair cDNA clone which encoded 18 amino acids that had high sequence homology with the carboxyl termini of other pepsinogens. Northern blot analysis revealed that this eDNA clone hybridized to a mRNA of 1,600 bases in the embryonic proventriculus but not to the mRNA in the adult proventriculus. The almost complete nucleotide sequence of embryonic chicken pepsinogen-cDNA was determined by sequenc ing longer cDNAs obtained by screening the same library with the 200-base pair eDNA and primer extension with a synthetic primer. The cDNA consisted of 1,281 nucleotides and encoded 383 amino acids for prepepsinogen. The predicted amino acid sequence was compared with the sequences of other aspartyl proteinases: pepsinogen A of human, monkey, pig, and chicken, progastricsin of monkey and rat, and bovine prochymosin. The phylogenetic tree constructed for them indicates the possibility that embryonic chicken pepsinogen diverged from prochymosin, after prochymosin and pepsinogen A had diverged from each other. -
Exogenous Enzymes in Animal Nutrition – Benefits and Limitations
Animal Nutrition and Feed Technology Volume 13 Number 3 September 2013 Special Issue EXOGENOUS ENZYMES IN ANIMAL NUTRITION – BENEFITS AND LIMITATIONS Guest Editor A.Z.M. Salem Facultad de Medicina Veterinaria y Zootecnia Universidad Autónoma del Estado de México, México and N. Odongo Animal Production and Health Section International Atomic Energy Agency, Vienna, Austria Animal Nutrition Association Indian Veterinary Research Institute, Izatnagar‐243 122, India www.anft.org Animal Nutrition and Feed Technology Volume 13 Number 3 September 2013 CONTENTS Special Issue: Exogenous Enzymes In Animal Nutrition - Benefits And Limitations Preface 34. A.Z.M. SALEM, N. ODONGO AND A.K. PATTANAIK Exogenous Enzymes in Animal Nutrition- Benefits and Limitations 335 In vivo Studies 35. D.B. DEAN, C.R. STAPLES, R.C. LITTELL, S. KIM AND A.T. ADESOGAN Effect of Method of Adding a Fibrolytic Enzyme to Dairy Cow Diets on Feed Intake Digestibility, Milk Production, Ruminal Fermentation, and Blood Metabolites 337 36. A. LARA BUENO, G.D. MENDOZA MARTÍNEZ, P.A. HERNÁNDEZ GARCÍA, J.A. MARTÍNEZ GARCÍA AND F.X. PLATA PÉREZ Evaluation of High Doses of Exogenous Fibrolytic Enzymes in Lambs Fed an Oat Straw Based Ration 355 37. O. ARCE-CERVANTES, G.D. MENDOZA, P.A. HERNÁNDEZ, M. MENESES, N. TORRES-SALADO AND O. LOERA The Effects of a Lignocellulolytic Extract of Fomes sp. EUM1 on the Intake, Digestibility, Feed Efficiency and Growth of Lambs 369 38. J.A. CAYETANO, A.Z.M. SALEM, B.M.A. MARIEZCURRENA, R. ROJO, M.A. CERRILLO-SOTO, H. GADO AND L.M. CAMACHO Effect of adding Salix babylonica Extracts and Exogenous Enzymes to Basal Diets on the Meat Quality of Growing Suffolk Lambs 373 39. -
Structure and Function of Plant Aspartic Proteinases
Eur. J. Biochem. 271, 2067–2075 (2004) Ó FEBS 2004 doi:10.1111/j.1432-1033.2004.04136.x REVIEW ARTICLE Structure and function of plant aspartic proteinases Isaura Simo˜ es and Carlos Faro Departamento de Biologia Molecular e Biotecnologia, Centro de Neurocieˆncias e Biologia Celular, Universidade de Coimbra and Departamento de Bioquı´mica, Faculdade de Cieˆncias e Tecnologia, Universidade de Coimbra, Portugal Aspartic proteinases of the A1 family are widely distributed processing and is absent from the mature form of the among plant species and have been purified from a variety enzyme. Its functions are still unclear but a role in the vac- of tissues. They are most active at acidic pH, are specifically uolar targeting of the precursors has been proposed. The inhibited by pepstatin A and contain two aspartic residues biological role of plant aspartic proteinases is also not indispensible for catalytic activity. The three-dimensional completely established. Nevertheless, their involvement in structure of two plant aspartic proteinases has been deter- protein processing or degradation under different conditions mined, sharing significant structural similarity with other and in different stages of plant development suggests some known structures of mammalian aspartic proteinases. With functional specialization. Based on the recent findings on the a few exceptions, the majority of plant aspartic proteinases diversity of A1 family members in Arabidopsis thaliana,new identified so far are synthesized with a prepro-domain and questions concerning novel structure–function relationships subsequently converted to mature two-chain enzymes. A among plant aspartic proteinases are now starting to be characteristic feature of the majority of plant aspartic pro- addressed. -
Mutational Analysis of Residues in Two Consensus Motifs in the Active Sites of Cathepsin El
J Biochem.132, 493-499 (2002) Mutational Analysis of Residues in Two Consensus Motifs in the Active Sites of Cathepsin El Jian Liu,*.•õ Takayuki Tsukuba,* Kuniaki Okamoto,* Masamichi Ohishi,•õ and Kenji Yamamoto*,2 Departments of Pharmacology and ttOral Surgery I, Graduate School of Dental Science, Kyushu University, Fukuoka 812-8582 Received June 19, 2002; accepted July 16, 2002 Cathepsin E, an intracellular aspartic proteinase of the pepsin family, is composed of two homologous domains, each containing the catalytic Asp residue in a consensus DTG motif. Here we examine the significance of residues in the motifs of rat cathepsin E by substitution of Asp98, Asp283, and Thr284 with other residues using site-directed muta genesis. Each of the mutant proenzymes, as well as the wild-type protein, was found in culture media and cell extracts when heterologously expressed in human embryonic kidney 293T cells. The single mutants D98A, D283A, and D283E, and the double mutants D98A/D283A and D98E/D283E showed neither autocatalytic processing nor enzymatic activities under acidic conditions. However, the D98E and T284S mutants retained the ability to transform into the mature forms, although they exhibited only about 13 and 40% of the activity of the wild-type enzyme, respectively. The Km values of these two mutants were similar to those of the wild-type enzyme, but their kcat values were greatly decreased. The K; values for pepstatin and the Ascaris pepsin inhibitor of the mutants and the wild-type enzyme were almost the same. The circular dichroism spectra of the two mutants were essentially the same as those of the wild-type enzyme at various pH values.