Stem-Like Cells and Glial Progenitors in the Adult Mouse Suprachiasmatic Nucleus
Total Page:16
File Type:pdf, Size:1020Kb
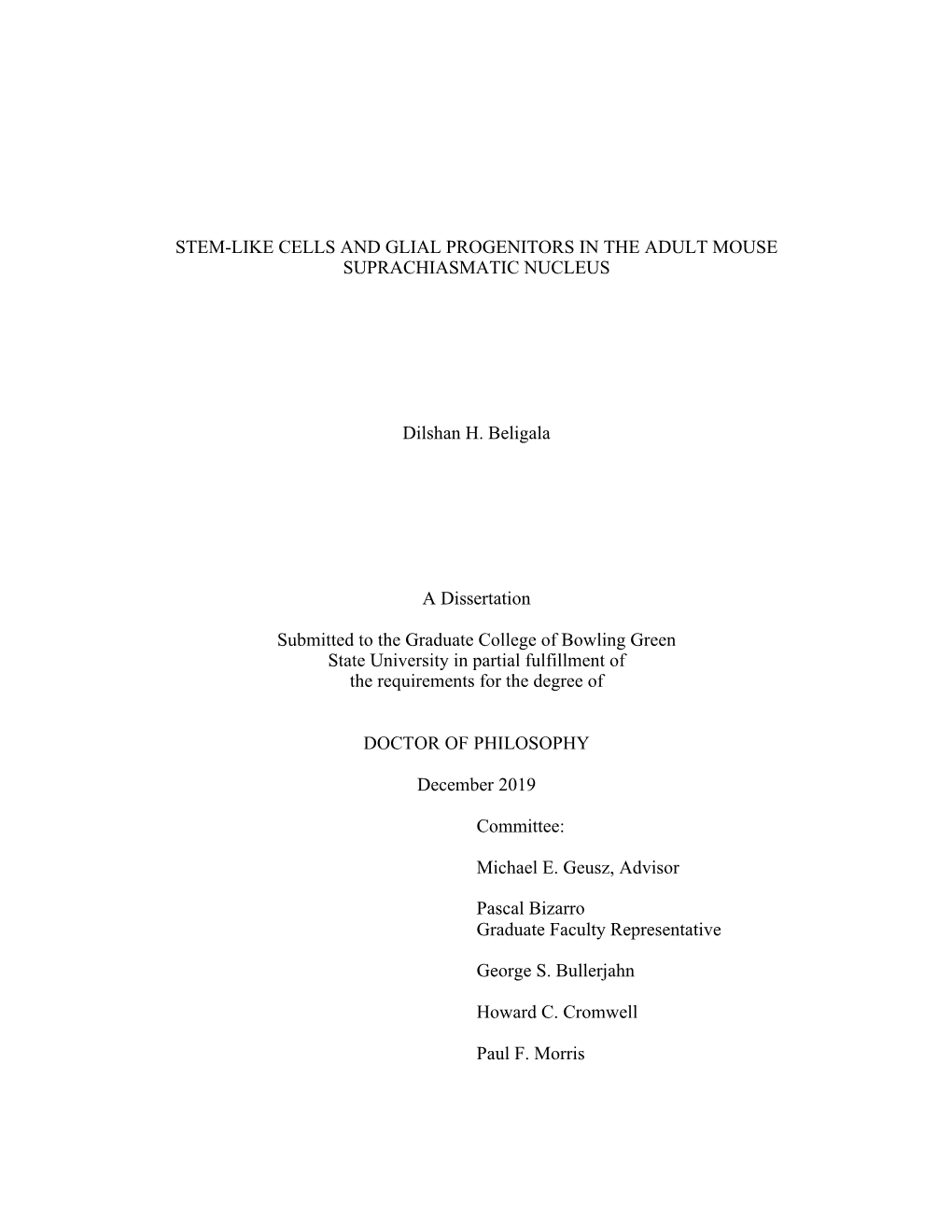
Load more
Recommended publications
-
Implications of Zonal Architecture on Differential Gene Expression
www.nature.com/scientificreports OPEN Implications of zonal architecture on diferential gene expression profling and altered pathway expressions in mandibular condylar cartilage Aisha M. Basudan1*, Mohammad Azhar Aziz2 & Yanqi Yang3 Mandibular condylar cartilage (MCC) is a multi-zonal heterogeneous fbrocartilage containing diferent types of cells, but the factors/mechanisms governing the phenotypic transition across the zones have not been fully understood. The reliability of molecular studies heavily rely on the procurement of pure cell populations from the heterogeneous tissue. We used a combined laser-capture microdissection and microarray analysis approach which allowed identifcation of diferential zone-specifc gene expression profling and altered pathways in the MCC of 5-week-old rats. The bioinformatics analysis demonstrated that the MCC cells clearly exhibited distinguishable phenotypes from the articular chondrocytes. Additionally, a set of genes has been determined as potential markers to identify each MCC zone individually; Crab1 gene showed the highest enrichment while Clec3a was the most downregulated gene at the superfcial layer, which consists of fbrous (FZ) and proliferative zones (PZ). Ingenuity Pathway Analysis revealed numerous altered signaling pathways; Leukocyte extravasation signaling pathway was predicted to be activated at all MCC zones, in particular mature and hypertrophic chondrocytes zones (MZ&HZ), when compared with femoral condylar cartilage (FCC). Whereas Superpathway of Cholesterol Biosynthesis showed predicted activation in both FZ and PZ as compared with deep MCC zones and FCC. Determining novel zone-specifc diferences of large group of potential genes, upstream regulators and pathways in healthy MCC would improve our understanding of molecular mechanisms on regional (zonal) basis, and provide new insights for future therapeutic strategies. -
CHD4/Nurd Complex Regulates Complement Gene Expression And
Shao et al. Clinical Epigenetics (2020) 12:31 https://doi.org/10.1186/s13148-020-00827-3 RESEARCH Open Access CHD4/NuRD complex regulates complement gene expression and correlates with CD8 T cell infiltration in human hepatocellular carcinoma Simin Shao1†, Haowei Cao1†, Zhongkun Wang1, Dongmei Zhou2, Chaoshen Wu1, Shu Wang1, Dian Xia1 and Daoyong Zhang1* Abstract Backgrounds: The NuRD (Nucleosome Remodeling and Deacetylation) complex is a repressive complex in gene transcription by modulating chromatin accessibility of target genes to transcription factors and RNA polymerase II. Although individual subunits of the complex have been implicated in many other cancer types, the complex’s role in human hepatocellular carcinoma (HCC) is not fully understood. More importantly, the NuRD complex has not yet been investigated as a whole in cancers. Methods: We analyzed the expression of the NuRD complex in HCC and evaluated the prognostic value of NuRD complex expression in HCC using the RNA-seq data obtained from the TCGA project. We examined the effect of CHD4 knockdown on HCC cell proliferation, apoptosis, migration, invasion, epithelial-mesenchymal transition, colony-forming ability, and on complement gene expression. We also performed bioinformatic analyses to investigate the correlation between the NuRD complex expression and immune infiltration. Results: We found that nine subunits, out of 14 subunits of the NuRD complex examined, were significantly overexpressed in HCC, and their expression levels were positively correlated with cancer progression. More importantly, our data also demonstrated that these subunits tended to be overexpressed as a whole in HCC. Subsequent studies demonstrated that knockdown of CHD4 in HCC cells inhibits cell proliferation, migration, invasion, and colony-forming ability and promotes apoptosis of HCC cells, indicating that the CHD4/NuRD complex plays oncogenic roles in HCC. -
Systematic Comparison of Sea Urchin and Sea Star Developmental Gene Regulatory Networks Explains How Novelty Is Incorporated in Early Development
ARTICLE https://doi.org/10.1038/s41467-020-20023-4 OPEN Systematic comparison of sea urchin and sea star developmental gene regulatory networks explains how novelty is incorporated in early development Gregory A. Cary 1,3,5, Brenna S. McCauley1,4,5, Olga Zueva1, Joseph Pattinato1, William Longabaugh2 & ✉ Veronica F. Hinman 1 1234567890():,; The extensive array of morphological diversity among animal taxa represents the product of millions of years of evolution. Morphology is the output of development, therefore phenotypic evolution arises from changes to the topology of the gene regulatory networks (GRNs) that control the highly coordinated process of embryogenesis. A particular challenge in under- standing the origins of animal diversity lies in determining how GRNs incorporate novelty while preserving the overall stability of the network, and hence, embryonic viability. Here we assemble a comprehensive GRN for endomesoderm specification in the sea star from zygote through gastrulation that corresponds to the GRN for sea urchin development of equivalent territories and stages. Comparison of the GRNs identifies how novelty is incorporated in early development. We show how the GRN is resilient to the introduction of a transcription factor, pmar1, the inclusion of which leads to a switch between two stable modes of Delta-Notch signaling. Signaling pathways can function in multiple modes and we propose that GRN changes that lead to switches between modes may be a common evolutionary mechanism for changes in embryogenesis. Our data additionally proposes a model in which evolutionarily conserved network motifs, or kernels, may function throughout development to stabilize these signaling transitions. 1 Department of Biological Sciences, Carnegie Mellon University, Pittsburgh, PA 15213, USA. -
Cyclin D1 Is a Direct Transcriptional Target of GATA3 in Neuroblastoma Tumor Cells
Oncogene (2010) 29, 2739–2745 & 2010 Macmillan Publishers Limited All rights reserved 0950-9232/10 $32.00 www.nature.com/onc SHORT COMMUNICATION Cyclin D1 is a direct transcriptional target of GATA3 in neuroblastoma tumor cells JJ Molenaar1,2, ME Ebus1, J Koster1, E Santo1, D Geerts1, R Versteeg1 and HN Caron2 1Department of Human Genetics, Academic Medical Center, University of Amsterdam, Amsterdam, The Netherlands and 2Department of Pediatric Oncology, Emma Kinderziekenhuis, Academic Medical Center, University of Amsterdam, Amsterdam, The Netherlands Almost all neuroblastoma tumors express excess levels of 2000). Several checkpoints normally prevent premature Cyclin D1 (CCND1) compared to normal tissues and cell-cycle progression and cell division. The crucial G1 other tumor types. Only a small percentage of these entry point is controlled by the D-type Cyclins that can neuroblastoma tumors have high-level amplification of the activate CDK4/6 that in turn phosphorylate the pRb Cyclin D1 gene. The other neuroblastoma tumors have protein. This results in a release of the E2F transcription equally high Cyclin D1 expression without amplification. factor that causes transcriptional upregulation of Silencing of Cyclin D1 expression was previously found to numerous genes involved in further progression of the trigger differentiation of neuroblastoma cells. Over- cell cycle (Sherr, 1996). expression of Cyclin D1 is therefore one of the most Neuroblastomas are embryonal tumors that originate frequent mechanisms with a postulated function in neuro- from precursor cells of the sympathetic nervous system. blastoma pathogenesis. The cause for the Cyclin D1 This tumor has a very poor prognosis and despite the overexpression is unknown. -
Nervous Tissue
Nervous Tissue Prof.Prof. ZhouZhou LiLi Dept.Dept. ofof HistologyHistology andand EmbryologyEmbryology Organization:Organization: neuronsneurons (nerve(nerve cells)cells) neuroglialneuroglial cellscells Function:Function: Ⅰ Neurons 1.1. structurestructure ofof neuronneuron somasoma neuriteneurite a.a. dendritedendrite b.b. axonaxon 1.11.1 somasoma (1)(1) nucleusnucleus LocatedLocated inin thethe centercenter ofof soma,soma, largelarge andand palepale--stainingstaining nucleusnucleus ProminentProminent nucleolusnucleolus (2)(2) cytoplasmcytoplasm (perikaryon)(perikaryon) a.a. NisslNissl bodybody b.b. neurofibrilneurofibril NisslNissl’’ss bodiesbodies LM:LM: basophilicbasophilic massmass oror granulesgranules Nissl’s Body (TEM) EMEM:: RERRER,, freefree RbRb FunctionFunction:: producingproducing thethe proteinprotein ofof neuronneuron structurestructure andand enzymeenzyme producingproducing thethe neurotransmitterneurotransmitter NeurofibrilNeurofibril thethe structurestructure LM:LM: EM:EM: NeurofilamentNeurofilament micmicrotubulerotubule FunctionFunction cytoskeleton,cytoskeleton, toto participateparticipate inin substancesubstance transporttransport LipofuscinLipofuscin (3)(3) CellCell membranemembrane excitableexcitable membranemembrane ,, receivingreceiving stimutation,stimutation, fromingfroming andand conductingconducting nervenerve impulesimpules neurite: 1.2 Dendrite dendritic spine spine apparatus Function: 1.3 Axon axon hillock, axon terminal, axolemma Axoplasm: microfilament, microtubules, neurofilament, mitochondria, -
Genetic Variability in the Italian Heavy Draught Horse from Pedigree Data and Genomic Information
Supplementary material for manuscript: Genetic variability in the Italian Heavy Draught Horse from pedigree data and genomic information. Enrico Mancin†, Michela Ablondi†, Roberto Mantovani*, Giuseppe Pigozzi, Alberto Sabbioni and Cristina Sartori ** Correspondence: [email protected] † These two Authors equally contributed to the work Supplementary Figure S1. Mares and foal of Italian Heavy Draught Horse (IHDH; courtesy of Cinzia Stoppa) Supplementary Figure S2. Number of Equivalent Generations (EqGen; above) and pedigree completeness (PC; below) over years in Italian Heavy Draught Horse population. Supplementary Table S1. Descriptive statistics of homozygosity (observed: Ho_obs; expected: Ho_exp; total: Ho_tot) in 267 genotyped individuals of Italian Heavy Draught Horse based on the number of homozygous genotypes. Parameter Mean SD Min Max Ho_obs 35,630.3 500.7 34,291 38,013 Ho_exp 35,707.8 64.0 35,010 35,740 Ho_tot 50,674.5 93.8 49,638 50,714 1 Definitions of the methods for inbreeding are in the text. Supplementary Figure S3. Values of BIC obtained by analyzing values of K from 1 to 10, corresponding on the same amount of clusters defining the proportion of ancestry in the 267 genotyped individuals. Supplementary Table S2. Estimation of genomic effective population size (Ne) traced back to 18 generations ago (Gen. ago). The linkage disequilibrium estimation, adjusted for sampling bias was also included (LD_r2), as well as the relative standard deviation (SD(LD_r2)). Gen. ago Ne LD_r2 SD(LD_r2) 1 100 0.009 0.014 2 108 0.011 0.018 3 118 0.015 0.024 4 126 0.017 0.028 5 134 0.019 0.031 6 143 0.021 0.034 7 156 0.023 0.038 9 173 0.026 0.041 11 189 0.029 0.046 14 213 0.032 0.052 18 241 0.036 0.058 Supplementary Table S3. -
KETCH1 Imports HYL1 to Nucleus for Mirna Biogenesis in Arabidopsis
KETCH1 imports HYL1 to nucleus for miRNA biogenesis in Arabidopsis Zhonghui Zhanga,b,1, Xinwei Guoa,c,1, Chunxiao Gea,1, Zeyang Maa, Mengqiu Jianga, Tianhong Lic, Hisashi Koiwad, Seong Wook Yange, and Xiuren Zhanga,2 aDepartment of Biochemistry and Biophysics, Institute for Plant Genomics and Biotechnology, Texas A&M University, College Station, TX 77843; bGuangdong Provincial Key Laboratory of Biotechnology for Plant Development, School of Life Science, South China Normal University, Guangzhou 510631, China; cCollege of Horticulture, China Agricultural University, Beijing 100193, China; dDepartment of Horticultural Sciences, Texas A&M University, College Station, TX 77843; and eDepartment of Systems Biology, College of Life Science and Biotechnology, Yonsei University, Seoul 120-749, Republic of Korea Edited by Xuemei Chen, University of California, Riverside, CA, and approved March 9, 2017 (received for review December 2, 2016) MicroRNA (miRNA) is processed from primary transcripts with hairpin premiRNAs in mammalians (11, 12). Importin-8 facilitates the structures (pri-miRNAs) by microprocessors in the nucleus. How recruitment of AGO2-containing RISC to target mRNAs to pro- cytoplasmic-borne microprocessor components are transported into mote efficient and specific gene silencing in the cytoplasm, whereas the nucleus to fulfill their functions remains poorly understood. Here, the protein can also transport AGO2 and AGO2 partners, GW we report KETCH1 (karyopherin enabling the transport of the proteins and miRNAs, into the nucleus to balance levels of cyto- cytoplasmic HYL1) as a partner of hyponastic leaves 1 (HYL1) protein, plasmic gene-silencing effectors (13–15). Arabidopsis encodes a core component of microprocessor in Arabidopsis and functional 18 importin β-proteins, among which few have also been reported counterpart of DGCR8/Pasha in animals. -
Homeobox Gene Expression Profile in Human Hematopoietic Multipotent
Leukemia (2003) 17, 1157–1163 & 2003 Nature Publishing Group All rights reserved 0887-6924/03 $25.00 www.nature.com/leu Homeobox gene expression profile in human hematopoietic multipotent stem cells and T-cell progenitors: implications for human T-cell development T Taghon1, K Thys1, M De Smedt1, F Weerkamp2, FJT Staal2, J Plum1 and G Leclercq1 1Department of Clinical Chemistry, Microbiology and Immunology, Ghent University Hospital, Ghent, Belgium; and 2Department of Immunology, Erasmus Medical Center, Rotterdam, The Netherlands Class I homeobox (HOX) genes comprise a large family of implicated in this transformation proces.14 The HOX-C locus transcription factors that have been implicated in normal and has been primarily implicated in lymphomas.15 malignant hematopoiesis. However, data on their expression or function during T-cell development is limited. Using degener- Hematopoietic cells are derived from stem cells that reside in ated RT-PCR and Affymetrix microarray analysis, we analyzed fetal liver (FL) in the embryo and in the adult bone marrow the expression pattern of this gene family in human multipotent (ABM), which have the unique ability to self-renew and thereby stem cells from fetal liver (FL) and adult bone marrow (ABM), provide a life-long supply of blood cells. T lymphocytes are a and in T-cell progenitors from child thymus. We show that FL specific type of hematopoietic cells that play a major role in the and ABM stem cells are similar in terms of HOX gene immune system. They develop through a well-defined order of expression, but significant differences were observed between differentiation steps in the thymus.16 Several transcription these two cell types and child thymocytes. -
A Computational Approach for Defining a Signature of Β-Cell Golgi Stress in Diabetes Mellitus
Page 1 of 781 Diabetes A Computational Approach for Defining a Signature of β-Cell Golgi Stress in Diabetes Mellitus Robert N. Bone1,6,7, Olufunmilola Oyebamiji2, Sayali Talware2, Sharmila Selvaraj2, Preethi Krishnan3,6, Farooq Syed1,6,7, Huanmei Wu2, Carmella Evans-Molina 1,3,4,5,6,7,8* Departments of 1Pediatrics, 3Medicine, 4Anatomy, Cell Biology & Physiology, 5Biochemistry & Molecular Biology, the 6Center for Diabetes & Metabolic Diseases, and the 7Herman B. Wells Center for Pediatric Research, Indiana University School of Medicine, Indianapolis, IN 46202; 2Department of BioHealth Informatics, Indiana University-Purdue University Indianapolis, Indianapolis, IN, 46202; 8Roudebush VA Medical Center, Indianapolis, IN 46202. *Corresponding Author(s): Carmella Evans-Molina, MD, PhD ([email protected]) Indiana University School of Medicine, 635 Barnhill Drive, MS 2031A, Indianapolis, IN 46202, Telephone: (317) 274-4145, Fax (317) 274-4107 Running Title: Golgi Stress Response in Diabetes Word Count: 4358 Number of Figures: 6 Keywords: Golgi apparatus stress, Islets, β cell, Type 1 diabetes, Type 2 diabetes 1 Diabetes Publish Ahead of Print, published online August 20, 2020 Diabetes Page 2 of 781 ABSTRACT The Golgi apparatus (GA) is an important site of insulin processing and granule maturation, but whether GA organelle dysfunction and GA stress are present in the diabetic β-cell has not been tested. We utilized an informatics-based approach to develop a transcriptional signature of β-cell GA stress using existing RNA sequencing and microarray datasets generated using human islets from donors with diabetes and islets where type 1(T1D) and type 2 diabetes (T2D) had been modeled ex vivo. To narrow our results to GA-specific genes, we applied a filter set of 1,030 genes accepted as GA associated. -
Downloaded and Searched Against the Dbest Database to Identify Ests
BMC Genomics BioMed Central Research article Open Access A transcription map of the 6p22.3 reading disability locus identifying candidate genes Eric R Londin1, Haiying Meng2 and Jeffrey R Gruen*2 Address: 1Graduate Program in Genetics, State University of New York at Stony Brook, NY, USA and 2Yale Child Health Research Center, Department of Pediatrics, Yale University School of Medicine, New Haven, CT, USA Email: Eric R Londin - [email protected]; Haiying Meng - [email protected]; Jeffrey R Gruen* - [email protected] * Corresponding author Published: 30 June 2003 Received: 22 April 2003 Accepted: 30 June 2003 BMC Genomics 2003, 4:25 This article is available from: http://www.biomedcentral.com/1471-2164/4/25 © 2003 Londin et al; licensee BioMed Central Ltd. This is an Open Access article: verbatim copying and redistribution of this article are permitted in all media for any purpose, provided this notice is preserved along with the article's original URL. reading disabilitydyslexia6p22.3In silicoESTs Abstract Background: Reading disability (RD) is a common syndrome with a large genetic component. Chromosome 6 has been identified in several linkage studies as playing a significant role. A more recent study identified a peak of transmission disequilibrium to marker JA04 (G72384) on chromosome 6p22.3, suggesting that a gene is located near this marker. Results: In silico cloning was used to identify possible candidate genes located near the JA04 marker. The 2 million base pairs of sequence surrounding JA04 was downloaded and searched against the dbEST database to identify ESTs. In total, 623 ESTs from 80 different tissues were identified and assembled into 153 putative coding regions from 19 genes and 2 pseudogenes encoded near JA04. -
Transcription Profiles of Age-At-Maturity-Associated Genes Suggest Cell Fate Commitment Regulation As a Key Factor in the Atlant
INVESTIGATION Transcription Profiles of Age-at-Maturity- Associated Genes Suggest Cell Fate Commitment Regulation as a Key Factor in the Atlantic Salmon Maturation Process Johanna Kurko,*,† Paul V. Debes,*,† Andrew H. House,*,† Tutku Aykanat,*,† Jaakko Erkinaro,‡ and Craig R. Primmer*,†,1 *Organismal and Evolutionary Biology Research Programme, University of Helsinki, Helsinki, Finland, 00014, †Institute of Biotechnology, University of Helsinki, Helsinki, Finland, 00014, and ‡Natural Resources Institute Finland (Luke), Oulu, Finland, 90014 ORCID IDs: 0000-0002-4598-116X (J.K.); 0000-0003-4491-9564 (P.V.D.); 0000-0001-8705-0358 (A.H.H.); 0000-0002-4825-0231 (T.A.); 0000-0002-7843-0364 (J.E.); 0000-0002-3687-8435 (C.R.P.) ABSTRACT Despite recent taxonomic diversification in studies linking genotype with phenotype, KEYWORDS follow-up studies aimed at understanding the molecular processes of such genotype-phenotype Atlantic salmon associations remain rare. The age at which an individual reaches sexual maturity is an important fitness maturation trait in many wild species. However, the molecular mechanisms regulating maturation timing process processes remain obscure. A recent genome-wide association study in Atlantic salmon (Salmo salar) vgll3 identified large-effect age-at-maturity-associated chromosomal regions including genes vgll3, akap11 mRNA and six6, which have roles in adipogenesis, spermatogenesis and the hypothalamic-pituitary-gonadal expression (HPG) axis, respectively. Here, we determine expression patterns of these genes during salmon de- cell fate velopment and their potential molecular partners and pathways. Using Nanostring transcription pro- regulation filing technology, we show development- and tissue-specific mRNA expression patterns for vgll3, akap11 and six6. Correlated expression levels of vgll3 and akap11, which have adjacent chromosomal location, suggests they may have shared regulation. -
Supplemental Materials ZNF281 Enhances Cardiac Reprogramming
Supplemental Materials ZNF281 enhances cardiac reprogramming by modulating cardiac and inflammatory gene expression Huanyu Zhou, Maria Gabriela Morales, Hisayuki Hashimoto, Matthew E. Dickson, Kunhua Song, Wenduo Ye, Min S. Kim, Hanspeter Niederstrasser, Zhaoning Wang, Beibei Chen, Bruce A. Posner, Rhonda Bassel-Duby and Eric N. Olson Supplemental Table 1; related to Figure 1. Supplemental Table 2; related to Figure 1. Supplemental Table 3; related to the “quantitative mRNA measurement” in Materials and Methods section. Supplemental Table 4; related to the “ChIP-seq, gene ontology and pathway analysis” and “RNA-seq” and gene ontology analysis” in Materials and Methods section. Supplemental Figure S1; related to Figure 1. Supplemental Figure S2; related to Figure 2. Supplemental Figure S3; related to Figure 3. Supplemental Figure S4; related to Figure 4. Supplemental Figure S5; related to Figure 6. Supplemental Table S1. Genes included in human retroviral ORF cDNA library. Gene Gene Gene Gene Gene Gene Gene Gene Symbol Symbol Symbol Symbol Symbol Symbol Symbol Symbol AATF BMP8A CEBPE CTNNB1 ESR2 GDF3 HOXA5 IL17D ADIPOQ BRPF1 CEBPG CUX1 ESRRA GDF6 HOXA6 IL17F ADNP BRPF3 CERS1 CX3CL1 ETS1 GIN1 HOXA7 IL18 AEBP1 BUD31 CERS2 CXCL10 ETS2 GLIS3 HOXB1 IL19 AFF4 C17ORF77 CERS4 CXCL11 ETV3 GMEB1 HOXB13 IL1A AHR C1QTNF4 CFL2 CXCL12 ETV7 GPBP1 HOXB5 IL1B AIMP1 C21ORF66 CHIA CXCL13 FAM3B GPER HOXB6 IL1F3 ALS2CR8 CBFA2T2 CIR1 CXCL14 FAM3D GPI HOXB7 IL1F5 ALX1 CBFA2T3 CITED1 CXCL16 FASLG GREM1 HOXB9 IL1F6 ARGFX CBFB CITED2 CXCL3 FBLN1 GREM2 HOXC4 IL1F7