Metabolic and Mechanistic Studies on Hydrazine Hepatotoxicity in The
Total Page:16
File Type:pdf, Size:1020Kb
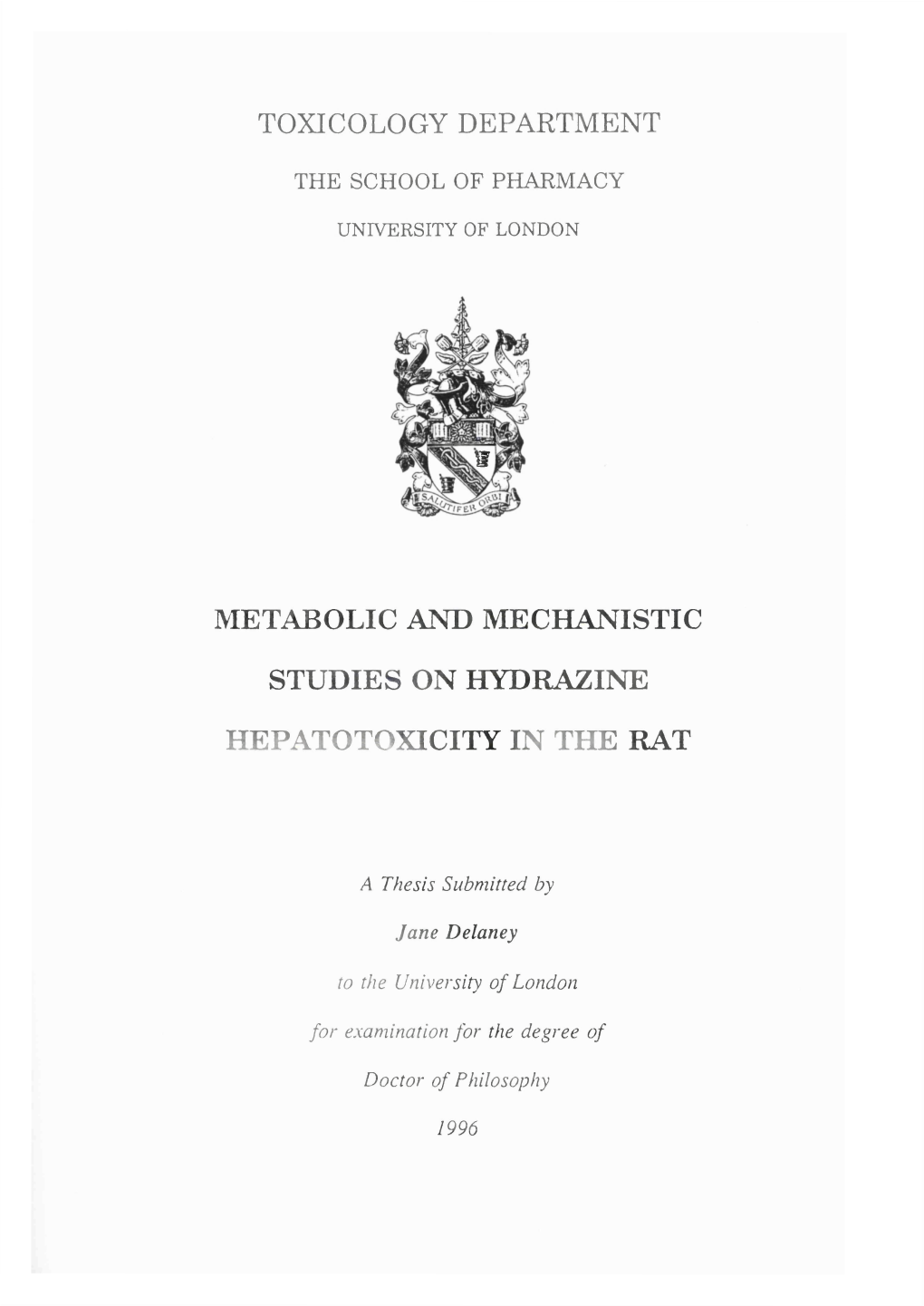
Load more
Recommended publications
-
XXXV International Congress of the European Association of Poisons Centres and Clinical Toxicologists (EAPCCT) 26–29 May 2015, St Julian's, Malta
Clinical Toxicology ISSN: 1556-3650 (Print) 1556-9519 (Online) Journal homepage: http://www.tandfonline.com/loi/ictx20 XXXV International Congress of the European Association of Poisons Centres and Clinical Toxicologists (EAPCCT) 26–29 May 2015, St Julian's, Malta To cite this article: (2015) XXXV International Congress of the European Association of Poisons Centres and Clinical Toxicologists (EAPCCT) 26–29 May 2015, St Julian's, Malta, Clinical Toxicology, 53:4, 233-403, DOI: 10.3109/15563650.2015.1024953 To link to this article: http://dx.doi.org/10.3109/15563650.2015.1024953 Published online: 26 Mar 2015. Submit your article to this journal Article views: 3422 View related articles View Crossmark data Citing articles: 2 View citing articles Full Terms & Conditions of access and use can be found at http://www.tandfonline.com/action/journalInformation?journalCode=ictx20 Download by: [UPSTATE Medical University Health Sciences Library] Date: 28 December 2016, At: 10:31 Clinical Toxicology (2015), 53, 233–403 Copyright © 2015 Informa Healthcare USA, Inc. ISSN: 1556-3650 print / 1556-9519 online DOI: 10.3109/15563650.2015.1024953 ABSTRACTS XXXV International Congress of the European Association of Poisons Centres and Clinical Toxicologists (EAPCCT) 26–29 May 2015, St Julian ’ s, Malta 1. Modelling dose-concentration-response Introduction: The American Association of Poison Control Cen- ters (AAPCC) published its fi rst annual report in 1983. Call data Ursula Gundert-Remy from sixteen US poison centers was chronicled in that report. Seven submitted data for the entire year. By July 2000, 63 centers Institute for Clinical Pharmacology and Toxicology, Charit é were part of the national poison center system, but only 59 submit- Medical School, Berlin, Germany ted data for the full year. -
Theoretical Problem Icho 2018
19th – 29th July 2018 Bratislava, SLOVAKIA Prague, CZECH REPUBLIC www.50icho.eu THEORETICAL PROBLEMS Country: Name as in passport: Student code: Language: 50th IChO 2018 International Chemistry Olympiad SLOVAKIA & CZECH REPUBLIC BACK TO WHERE IT ALL BEGAN XXX-X INTERNATIONAL CHEMISTRY OLYMPIAD / SLOVAKIA & CZECH REPUBLIC, 2018 Table of Contents Instructions ...................................................................................................................................... 2 Physical constants and equations ................................................................................................... 3 Problem 1. DNA .............................................................................................................................. 5 Problem 2. Repatriation of remains in the middle ages .................................................................. 14 Problem 3. Emerging electro-mobility ............................................................................................ 22 Problem 4. Column chromatography of radioactive copper ........................................................... 30 Problem 5. Bohemian garnet ......................................................................................................... 34 Problem 6. Let’s go mushrooming ................................................................................................. 41 Problem 7. Cidofovir ...................................................................................................................... 47 -
Pyridoxine in Clinical Toxicology: a Review Philippe Lheureux, Andrea Penaloza and Mireille Gris
78 Review Pyridoxine in clinical toxicology: a review Philippe Lheureux, Andrea Penaloza and Mireille Gris Pyridoxine (vitamin B6) is a co-factor in many enzymatic controversial. This paper reviews the various indications pathways involved in amino acid metabolism: the main of pyridoxine in clinical toxicology and the supporting biologically active form is pyridoxal 5-phosphate. literature. The potential adverse effects of excessive Pyridoxine has been used as an antidote in acute pyridoxine dosage will also be summarized. intoxications, including isoniazid overdose, Gyromitra European Journal of Emergency Medicine 12:78–85 mushroom or false morrel (monomethylhydrazine) c 2005 Lippincott Williams & Wilkins. poisoning and hydrazine exposure. It is also recommended as a co-factor to improve the conversion of glyoxylic acid European Journal of Emergency Medicine 2005, 12:78–85 into glycine in ethylene glycol poisoning. Other indications Keywords: Antidotes, crimidin, drug-induced neuropathy, ethylene glycol, are recommended by some sources (for example crimidine hydrazine, isoniazid, metadoxine, pyridoxine poisoning, zipeprol and theophylline-induced seizures, adjunct to d-penicillamine chelation), without significant Department of Emergency Medicine, Erasme University Hospital, Brussels, Belgium. supporting data. The value of pyridoxine or its congener Correspondence to Philippe Lheureux, Department of Emergency Medicine, metadoxine as an agent for hastening ethanol metabolism Erasme University Hospital, 808 route de Lennik, 1070 Brussels, Belgium. or improving vigilance in acute alcohol intoxication is E-mail: [email protected] Introduction ing. More controversial issues include alcohol intoxication Pyridoxine or vitamin B6 is a highly water-soluble and zipeprol or theophylline-induced seizures. vitamin. Its main biologically active form is a phosphate ester of its aldehyde form, pyridoxal 5-phosphate (P5P). -
Gyromitrin Poisoning: More Questions Than Answers
Gyromitrin poisoning: more questions than answers Denis R. Benjamin, MD some dedicated mycophagist, who had Many of these clues to the toxin never eaten the mushroom for many years made any coherent sense, even though it “It is perhaps ironic for a mushroom, without any ill effects, would suddenly was known for some years that the toxins Gyromitra esculenta, whose very name and unaccountably take ill. This too could be destroyed by cooking.” (From means edible, to be so poisonous under was passed off as the development of an Benjamin, 1995.) certain circumstances. Surprisingly, allergy in the unfortunate individual, the toxins were only characterized as that the mushrooms had been mistaken ll the enigmas related to this recently as 1968. A number of factors for a poisonous variety, or a rotten toxin remain unresolved. The conspired against the investigators of this batch had been eaten. To compound the current literature merely repeats mushroom poison (Lincoff and Mitchel, difficulties, Gyromitra esculenta caused Awhat was published before 1990. A 1977). The first was the observation that many poisonings in Europe, while in the deluge of “cut and paste.” No meaningful only a few of the participants eating the western USA, the seemingly identical research has been done in the past same quantity of the same mushroom species appeared largely harmless. All three decades. This is due to a number would become ill. Because of this, the sorts of explanations were proposed of factors. The first was the demise of poisoning was immediately ascribed to to explain this discrepancy, including academic pharmacognosy departments, ‘allergy’ or ‘individual idiosyncrasy.’ The such fanciful ones as suggesting that responsible for investigating the biology next problematic observation was that Americans cook their vegetables better. -
Synthetic and Naturally Occurring Hydrazines As Possible Cancer Causative Agents
[CANCER RESEARCH 35, 3693-3697 December 1975] Synthetic and Naturally Occurring Hydrazines as Possible Cancer Causative Agents Bela Toth' The Eppley Institute for Research in Cancer, University of Nebraska Medical Center, Omaha, Nebraska 68105 SUMMARY SYNTHETIC HYDRAZINES The various synthetic substituted hydrazines, which cause tumors in animals, are briefly enumerated. To date, 19 of Studies on the carcinogenic potentialities of synthetic them have proved to be tumorigenic in animals. A number substituted hydrazines began in 1962, when it was shown of these chemicals are found today in the environment, in that the base compound hydrazine sulfate induced lung industry, in agriculture, and in medicine, and the human neoplasms in mice (1). Subsequently, a series of hydrazine population is exposed to a certain degree to some of them. derivatives were investigated in various laboratories for Hydrazine also occurs in nature in tobacco and tobacco tumor-inducing capabilities. These studies clearly demon smoke. The three other naturally occurring hydrazine strated that these chemicals are indeed powerful tumori compounds are N-methyl-N-formylhydrazine, which oc genic substances in mice, hamsters, and rats, due to their curs in the wild edible mushroom, Gyromitra esculenta, tumor-inducing abilities in the intestines, brain, lungs, and @-N-[―y-L(+)-glutamylJ-4-hydroxymethylphenyl blood vessels, liver, breasts, kidneys, etc. Now, we know of hydrazine and 4-hydroxymethylphenylhydrazine, whkh 19 hydrazine derivatives that have been shown to be tumor are found in the commonly eaten cultivated mushroom, inducers. These include, in addition to hydrazine (1, 32), Agaricus bisporus. Tumorigenesis studies with the natu methyl- (35, 40), 1,2-dimethyl- (6, 27, 36, 46, 52), 1,1- rally occurring hydrazines are in progress. -
Hydrazine 1 Hydrazine
Hydrazine 1 Hydrazine Hydrazine Identifiers [1] CAS number 302-01-2 , 7803-57-8 (hydrate) [2] EC number 206-114-9 UN number 2029 (anhydrous) 2030 (aq. soln., 37–64%) 3293 (aq. soln., <37%) RTECS number MU7175000 Properties Molecular formula N H 2 4 Molar mass 32.05 g/mol (anhydrous) 50.06 g/mol (hydrate) Appearance Colourless liquid Density 1.0045 g/cm3 (anhydrous) 1.032 g/cm3 (hydrate) Melting point 1 °C (274 K, anhydrous) -51.7 °C (hydrate) Boiling point 114 °C (387 K), anhydrous 119 °C (hydrate) Solubility in water miscible Acidity (pK ) 8.1 a Refractive index (n ) [3] D 1.46044 (22 °C, anhydrous) 1.4284 (hydrate) Viscosity 0.876 cP (25 °C) Structure Hydrazine 2 Molecular shape pyramidal at N [4] Dipole moment 1.85 D Hazards [5] MSDS ICSC 0281 EU Index 007-008-00-3 EU classification Carc. Cat. 2 Toxic (T) Corrosive (C) Dangerous for the environment (N) R-phrases R45, R10, R23/24/25, R34, R43, R50/53 S-phrases S53, S45, S60, S61 NFPA 704 Flash point 52 °C Autoignition 24–270 °C (see text) temperature Explosive limits 1.8–100% LD [6] 50 59–60 mg/kg (oral in rats, mice) Related compounds Related nitrogen hydrides Ammonia Hydrazoic acid Related compounds monomethylhydrazine dimethylhydrazine phenylhydrazine [7] (what is this?) (verify) Except where noted otherwise, data are given for materials in their standard state (at 25 °C, 100 kPa) Infobox references Hydrazine is an inorganic chemical compound with the formula N H . It is a colourless liquid with an 2 4 ammonia-like odor and is derived from the same industrial chemistry processes that manufacture ammonia. -
Toxic Fungi of Western North America
Toxic Fungi of Western North America by Thomas J. Duffy, MD Published by MykoWeb (www.mykoweb.com) March, 2008 (Web) August, 2008 (PDF) 2 Toxic Fungi of Western North America Copyright © 2008 by Thomas J. Duffy & Michael G. Wood Toxic Fungi of Western North America 3 Contents Introductory Material ........................................................................................... 7 Dedication ............................................................................................................... 7 Preface .................................................................................................................... 7 Acknowledgements ................................................................................................. 7 An Introduction to Mushrooms & Mushroom Poisoning .............................. 9 Introduction and collection of specimens .............................................................. 9 General overview of mushroom poisonings ......................................................... 10 Ecology and general anatomy of fungi ................................................................ 11 Description and habitat of Amanita phalloides and Amanita ocreata .............. 14 History of Amanita ocreata and Amanita phalloides in the West ..................... 18 The classical history of Amanita phalloides and related species ....................... 20 Mushroom poisoning case registry ...................................................................... 21 “Look-Alike” mushrooms ..................................................................................... -
Hypoglycemia and Death in Mice Following Experimental Exposure to an Extract of Trogia Venenata Mushrooms
Hypoglycemia and Death in Mice Following Experimental Exposure to an Extract of Trogia venenata Mushrooms GuoQing Shi1., Jun He2., Tao Shen1, Robert E. Fontaine1,3, Lin Yang4, ZhongYu Zhou5, Hong Gao2, YanFeng Xu2, Chuan Qin2, ZhuLiang Yang5, JiKai Liu5, WenLi Huang4*, Guang Zeng1* 1 Chinese Field Epidemiology Training Program, Chinese Center for Disease Control and Prevention, Beijing, China, 2 The Institute of Laboratory Animal Science, Chinese Academy of Medical Sciences and Peking Union Medical College, Beijing, China, 3 United States Centers for Disease Control and Prevention, Atlanta, Georgia, United States of America, 4 Yunnan Institute of Endemic Diseases Control and Prevention, Dali, Yunnan Province, China, 5 State Key Laboratory of Phytochemistry and Plant Resources in West China, Kunming Institute of Botany, Chinese Academy of Sciences, Kunming, Yunnan Province, China Abstract Background: Clusters of sudden unexplained death (SUD) in Yunnan Province, China, have been linked to eating Trogia venenata mushrooms. We evaluated the toxic effect of this mushroom on mice. Methods: We prepared extracts of fresh T. venenata and Laccaria vinaceoavellanea mushrooms collected from the environs of a village that had SUD. We randomly allocated mice into treatment groups and administered mushroom extracts at doses ranging from 500 to 3500 mg/kg and water (control) via a gavage needle. We observed mice for mortality for 7 days after a 3500 mg/kg dose and for 24 hours after doses from 500 to 3000 mg/kg. We determined biochemical markers from serum two hours after a 2000 mg/kg dose. Results: Ten mice fed T. venenata extract (3500 mg/kg) died by five hours whereas all control mice (L. -
Poisoning Due to Raw Gyromitra Esculenta (False Morels) West of the Rockies
CASE REPORT • OBSERVATIONS DE CAS Poisoning due to raw Gyromitra esculenta (false morels) west of the Rockies Anne M. Leathem, BSP, MSP;* Thomas J. Dorran, MD, MBA† ABSTRACT Vomiting with abdominal pain is a common presentation in the emergency department (ED). Without a careful history, unusual causes, such as toxic ingestion, may evade diagnosis. We report a case of an Asian couple who presented to the ED with vomiting and epigastric distress. They were discharged with no definite diagnosis, but on a return ED visit the following day were diag- nosed with toxic ingestion of Gyromitra esculenta, commonly known as the western false morel. The patients were admitted and treated with intravenous hydration and pyridoxine. Both patients developed mild hepatotoxicity but went on to fully recover. This case demonstrates that the west- ern false morel may cause significant toxicity and it highlights the importance of obtaining a com- plete history in patients who present with non-specific gastrointestinal symptoms. Key words: Gyromitra esculenta, false morel, gyromitrin, monomethylhydrazine, mushroom poisoning RÉSUMÉ Le service d’urgence reçoit souvent des patients qui présentent douleurs abdominales et vomisse- ments. Sans anamnèse, des causes inhabituelles, telle que l’ingestion toxique, peuvent échapper au diagnostic. Nous rapportons le cas d’un couple asiatique qui s’est présenté au service d’urgence en détresse épigastrique accompagnée de vomissements. Le couple a reçu son congé sans diagnostic précis, mais s’est présenté à nouveau le lendemain à l’urgence, où on a alors diagnostiqué une in- gestion toxique de Gyromitra esculenta, communément appelé fausse morille. Les patients ont été admis et traités par hydratation et pyridoxine intraveineuses. -
Mushroom Poisoning
CHAPTER Mushroom Poisoning 114 Bhupen Barman, KG Lynrah, Iadarilang Tiewsoh INTRODUCTION Acute gastroenteritis – A wide variety of non eatable Mushroom poisoning among other forms of poisoning mushrooms when consumed causes nausea, vomiting, contributes to high morbidity and mortality in the diarrhea and abdominal cramps within one to three country. In certain ethnic populations of India, mushroom hours of consumption. These symptoms are caused most is an important constituent of their diet. The incidence of commonly by the chlorophyllum molybdites species are mushroom poisoning in India in the recent years has been also called “backyard mushrooms”. recognized due to increasing awareness and the affected NEUROTOXIC SYNDROMES individuals seeking health care at the earliest. The tropical a. The hallucinogenic effects are produced by the belt of the country with its biodiversity is a mother load ingestion of mushroom containing psilocybin of different fungal mushroom species. As per studies and psilocin. They are known to be abused for conducted in India, there are 1200 species with only 50 recreational purposes. to 100 species known to be poisonous. Twelve groups of the identified mushroom toxins have been identified b. CNS excitation and depression: This syndrome responsible for 14 described clinical syndromes. is caused by Amanita species containing toxins muscimol and ibotenic acid. Muscimol is a CNS EPIDEMIOLOGY depressant whereas ibotenic acid has excitatory From the studies conducted the 50-100 toxic mushrooms effects at glutamic acid receptors in the CNS. produces the mycotoxins responsible for different clinical Symptoms include somnolence, dizziness, syndromes which are described below. In the United hallucination, dysphoria, bizarre behavior and States the North American mycological association seizures. -
Toxic Agitation and Seizures
Toxic Agitation and Seizures Dr. Caitlin Wolfe Dalhousie Spring Refresher April 2021 Pathophysiology of seizures from toxic exposures Learning List common xenobiotics Objectives associated with seizures Approach to the seizing toxicology patient • No financial disclosures • Emergency Physician at the Halifax Infirmary • Medical Toxicologist at the IWK Regional Poison Control Centre Disclosures and • Member of the Health Canada network, Affiliations the Canadian Surveillance System for Poison Information (CSSPI) • Assistant Professor, Department of Emergency Medicine, Dalhousie University • Agitation and delirium are features of numerous toxidromes • Anticholinergics, Serotonin syndrome, Sympathomimetics, withdrawal from ethanol or sedative-hypnotics “Toxic • Pitfalls and risks: • Medical decompensation: hyperthermia, agitation” rhabdomyolysis, arrhythmias… • Behaviour dangerous to self and others • Missed medical diagnoses (long overlap of DDx) • Missed psychiatric diagnoses • Missed physical injuries • Seizures are the most extreme form • Case series of first seizures = 6-47% are from toxic or metabolic causes • Seizures can be from: • Acute ingestion Seizures from Toxic • Withdrawal Causes • Chronic use • Drug-drug interactions • Idiosyncratic • Tramadol, Buproprion • Dose dependent • Theophylline • Enhanced excitatory neurotransmission (glutamate and NMDA) and/or inhibition of GABAergic inhibitory tone • 4 main direct mechanisms Pathophysiology of • Activity at NMDA and GABA receptors Toxin Related • Alteration of neuronal resting potential -
Download MSDS File
Safety Data Sheet - Version 5.0 Preparation Date 8/27/2015 Latest Revision Date (If Revised) SDS Expiry Date 8/25/2018 1. IDENTIFICATION OF THE SUBSTANCE/MIXTURE AND OF THE COMPANY/UNDERTAKING 1.1 Product Identifier Chemical Name Gyromitrin Catalogue # G931900 1.2 Relevant Identified Uses of the Substance or Mixture and Uses Advised Against Product Uses To be used only for scientific research and development. Not for use in humans or animals. 1.3 Details of the Supplier of the Safety Data Sheet Company Toronto Research Chemicals 2 Brisbane Road Toronto, ON M3J 2J8 CANADA Telephone +14166659696 FAX +14166654439 Email [email protected] 1.4 Emergency Telephone Number Emergency# +14166659696 between 0800-1700 (GMT-5) 2. HAZARDS IDENTIFICATION WHMIS Classification (Canada) WHMIS Symbols (Canada) None Not WHMIS controlled. 2.1/2.2 Classification of the Substance or Mixture and Label Elements GHS Hazards Classification (According to EU Regulation 1272/2008 and US OSHA 1910.1200) Not a hazardous substance by GHS. EU Classification (According to EU Regulation 67/548/EEC) Not a hazardous substance by this Classification. EU Risk and Safety Statements (According to EU Regulation 67/548/EEC) Hazard Statements Hazard Codes None Risk Codes and Phrases None Not a hazardous substance by this Classification. Safety Precaution Codes and Phrases GHS Hazards Identification (According to EU Regulation 1272/2008 and US OSHA 1910.1200) Signal Word None GHS Hazard Statements None Not a hazardous substance according to GHS. Toronto Research Chemicals - G931900 Page 1 This Safety Data Sheet contains 16 sections. All 16 sections must be present for this document to be valid.