1 Air Quality Impacts of Increased Use of Ethanol Under The
Total Page:16
File Type:pdf, Size:1020Kb
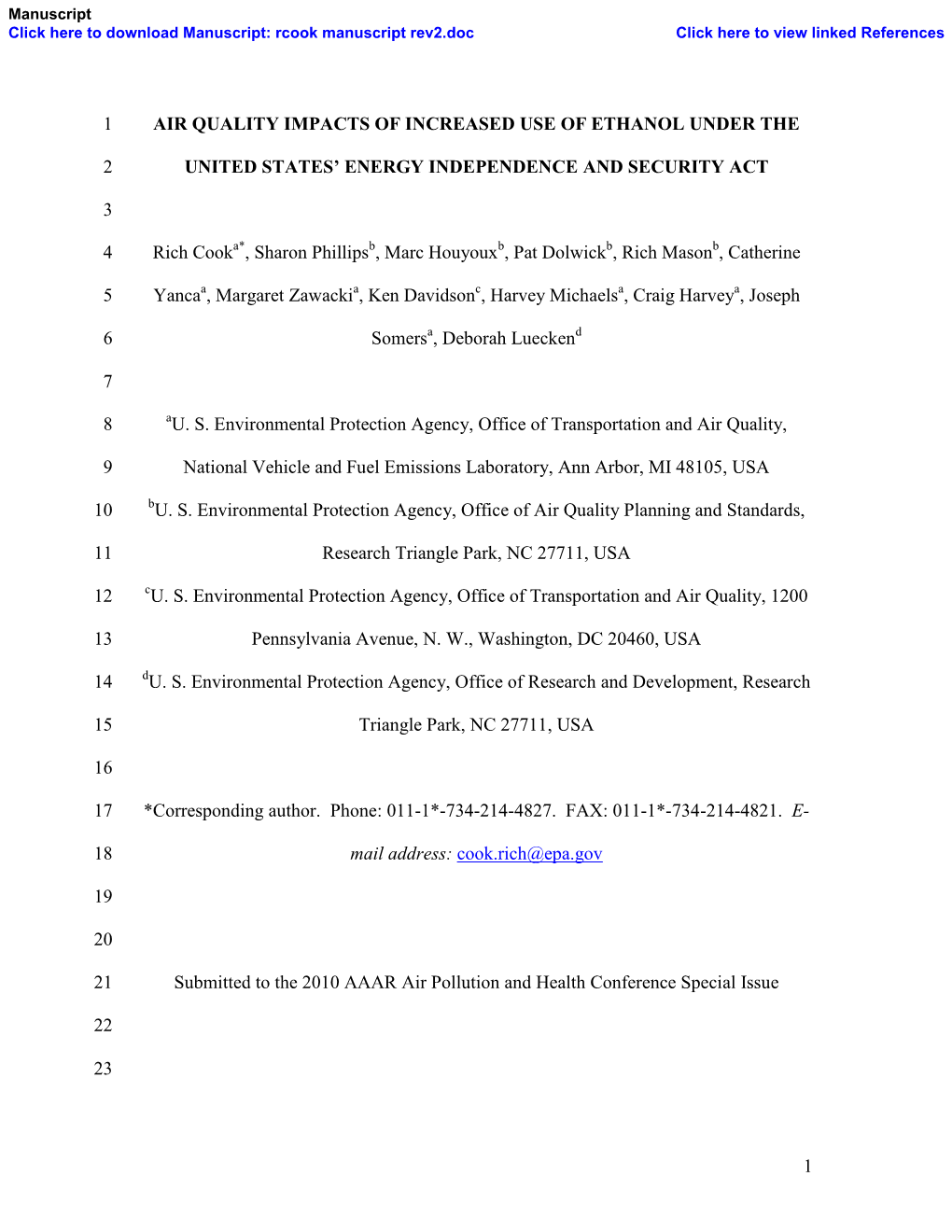
Load more
Recommended publications
-
Ozonedisinfection.Pdf
ETI - Environmental Technology Initiative Project funded by the U.S. Environmental Protection Agency under Assistance Agreement No. CX824652 What is disinfection? Human exposure to wastewater discharged into the environment has increased in the last 15 to 20 years with the rise in population and the greater demand for water resources for recreation and other purposes. Disinfection of wastewater is done to prevent infectious diseases from being spread and to ensure that water is safe for human contact and the environment. There is no perfect disinfectant. However, there are certain characteristics to look for when choosing the most suitable disinfectant: • Ability to penetrate and destroy infectious agents under normal operating conditions; • Lack of characteristics that could be harmful to people and the environment; • Safe and easy handling, shipping, and storage; • Absence of toxic residuals, such as cancer-causing compounds, after disinfection; and • Affordable capital and operation and maintenance (O&M) costs. What is ozone disinfection? One common method of disinfecting wastewater is ozonation (also known as ozone disinfection). Ozone is an unstable gas that can destroy bacteria and viruses. It is formed when oxygen molecules (O2) collide with oxygen atoms to produce ozone (O3). Ozone is generated by an electrical discharge through dry air or pure oxygen and is generated onsite because it decomposes to elemental oxygen in a short amount of time. After generation, ozone is fed into a down-flow contact chamber containing the wastewater to be disinfected. From the bottom of the contact chamber, ozone is diffused into fine bubbles that mix with the downward flowing wastewater. See Figure 1 on page 2 for a schematic of the ozonation process. -
Ethanol Purification with Ozonation, Activated Carbon Adsorption, and Gas Stripping Shinnosuke Onuki Iowa State University
Agricultural and Biosystems Engineering Agricultural and Biosystems Engineering Publications 9-4-2015 Ethanol purification with ozonation, activated carbon adsorption, and gas stripping Shinnosuke Onuki Iowa State University Jacek A. Koziel Iowa State University, [email protected] William S. Jenks Iowa State University, [email protected] Lingshuang Cai Iowa State University Somchai Rice Iowa State University SeFoe nelloxtw pa thige fors aaddndition addal aitutionhorsal works at: https://lib.dr.iastate.edu/abe_eng_pubs Part of the Agriculture Commons, Bioresource and Agricultural Engineering Commons, and the Organic Chemistry Commons The ompc lete bibliographic information for this item can be found at https://lib.dr.iastate.edu/ abe_eng_pubs/909. For information on how to cite this item, please visit http://lib.dr.iastate.edu/ howtocite.html. This Article is brought to you for free and open access by the Agricultural and Biosystems Engineering at Iowa State University Digital Repository. It has been accepted for inclusion in Agricultural and Biosystems Engineering Publications by an authorized administrator of Iowa State University Digital Repository. For more information, please contact [email protected]. Ethanol purification with ozonation, activated carbon adsorption, and gas stripping Abstract Fermentation of sugar to produce ethanol also produces volatile byproducts. This study was aimed at purifying corn-based ethanol for industrial and pharmaceutical use. The er search was on treatment for 10 impurities removal after distillation. The the anol headspace was sampled with solid-phase microextraction and analyzed with gas chromatography–mass spectrometry. A 40 mg/L ozone treatment resulted in >56% and >36% removal of styrene and 2-pentylfuran, respectively, without significant generation of byproducts. -
Publication No. 17: Ozone Treatment of Private Drinking Water Systems
PRIVATE DRINKING WATER IN CONNECTICUT Publication Date: April 2009 Publication No. 17: Ozone Treatment of Private Drinking Water Systems Effective Against: Pathogenic (disease-causing) organisms including bacteria and viruses, phenols (aromatic organic compounds), some color, taste and odor problems, iron, manganese, and turbidity. Not Effective Against: Large cysts and some other large organisms resulting from possible or probable sewage contamination, inorganic chemicals, and heavy metals. How Ozone (O3) Treatment Works Ozone is a chemical form of pure oxygen. Like chlorine, ozone is a strong oxidizing agent and is used in much the same way to kill disease-causing bacteria and viruses. It is effective against most amoebic cysts, and destroys bacteria and some aromatic organic compounds (such as phenols). Ozone may not kill large cysts and some other large organisms, so these should be eliminated by filtration or other procedures prior to ozone treatment. Ozone is effective in eliminating or controlling color, taste, and odor problems. It also oxidizes iron and manganese. Ozone treatment units are installed as point-of-use treatment systems. Raw water enters one opening and treated water emerges from another. Inside the treatment unit, ozone is produced by an electrical corona discharge or ultraviolet irradiation of dry air or oxygen. The ozone is mixed with the water whenever the water pump is running. Ozone generation units require a system to clean and remove the humidity from the air. For proper disinfection the water to be treated must have negligible color and turbidity levels. The system requires routine maintenance and an ozone treatment system can be very energy consumptive. -
Interaction of Ozone and Hydrogen Peroxide in Water Implications For
UC Irvine Faculty Publications Title Interaction of ozone and hydrogen peroxide in water: Implications for analysis of H 2 O 2 in air Permalink https://escholarship.org/uc/item/7j7454z7 Journal Geophysical Research Letters, 9(3) ISSN 00948276 Authors Zika, R. G Saltzman, E. S Publication Date 1982-03-01 DOI 10.1029/GL009i003p00231 License https://creativecommons.org/licenses/by/4.0/ 4.0 Peer reviewed eScholarship.org Powered by the California Digital Library University of California GEOPHYSICLARESEARCH LETTERS, VOL. 9, NO. 3, PAGES231-234 , MARCH1982 INTERACTION OF OZONE AND HYDROGEN PEROXIDE IN WATER: IMPLICATIONSFORANALYSIS oFH20 2 IN AIR R.G. Zika and E.S. Saltzman Division of Marine and Atmospheric Chemistry, University of Miami, Miami, Florida 331#9 Abstract. We have attempted to measure gaseous Analytical Methods H202 in air usingan aqueoustrapping method. With continuousbubbling, H 20 2 levels in the traps reacheda a. Hydrogen Peroxide. Hydrogen peroxide in aqueous plateau, indicating that a state of dynamic equilibrium solution was measured using a modified fluorescence involving H202 destrbction was established. We decay technique [Perschke and Broda, 1976; Zika and attribute this behavior to the interaction of ozone and its Zelmer, 1982]. The method involved the addition of a decompositionproducts (OH, O[) withH 20 2 inacld:•ous known amot•at of scopoletin (6-methyl-7-hydroxyl-i,2- solution. This hypothesis was investigated by replacing benzopyrone)to a pH 7.0 phosphatebuffered. sample. the air stream with a mixture of N2, 02 and 0 3. The The sample was prepared bY diluting an aliquot of the results Of this experiment show that H O was both reaction solution to 20 mls with low contaminant producedand destroyedin the traps. -
Nitrogen Oxides
Pollution Prevention and Abatement Handbook WORLD BANK GROUP Effective July 1998 Nitrogen Oxides Nitrogen oxides (NOx) in the ambient air consist 1994). The United States generates about 20 mil- primarily of nitric oxide (NO) and nitrogen di- lion metric tons of nitrogen oxides per year, about oxide (NO2). These two forms of gaseous nitro- 40% of which is emitted from mobile sources. Of gen oxides are significant pollutants of the lower the 11 million to 12 million metric tons of nitrogen atmosphere. Another form, nitrous oxide (N2O), oxides that originate from stationary sources, is a greenhouse gas. At the point of discharge about 30% is the result of fuel combustion in large from man-made sources, nitric oxide, a colorless, industrial furnaces and 70% is from electric utility tasteless gas, is the predominant form of nitro- furnaces (Cooper and Alley 1986). gen oxide. Nitric oxide is readily converted to the much more harmful nitrogen dioxide by Occurrence in Air and Routes of Exposure chemical reaction with ozone present in the at- mosphere. Nitrogen dioxide is a yellowish-or- Annual mean concentrations of nitrogen dioxide ange to reddish-brown gas with a pungent, in urban areas throughout the world are in the irritating odor, and it is a strong oxidant. A por- range of 20–90 micrograms per cubic meter (µg/ tion of nitrogen dioxide in the atmosphere is con- m3). Maximum half-hour values and maximum 24- verted to nitric acid (HNO3) and ammonium hour values of nitrogen dioxide can approach 850 salts. Nitrate aerosol (acid aerosol) is removed µg/m3 and 400 µg/m3, respectively. -
Reducing Nox Emissions on the Road
EUROPEAN CONFERENCE OF MINISTERS OF TRANSPORT REDUCING NOX EMISSIONS ON THE ROAD ENSURING FUTURE EXHAUSTS EMISSION LIMITS DELIVER AIR QUALITY STANDARDS Reducing NOx Emissions on the Road FOREWORD AND ACKNOWLEDGEMENTS Transport Ministers noted the conclusions and recommendations of this report at the meeting of the Council of the ECMT in Dublin on 17-18 May 2006, and asked the Secretariat to transmit the report to the UN/ECE with a request to expedite deliberations on improved vehicle certification tests for NOx emissions for adoption world-wide. This was duly done. The ECMT is grateful to Heinz Steven of the RWTÜV Institute for Vehicle Technology in Germany for the analysis presented in this paper. The report was prepared by the ECMT Group on Transport and the Environment in co-operation with the OECD Environment Policy Committee’s Working Group on Transport. © ECMT, 2006 1 Reducing NOx Emissions on the Road TABLE OF CONTENTS ACKNOWLEDGEMENTS ...............................................................................................................1 EXECUTIVE SUMMARY ................................................................................................................3 1. INTRODUCTION .....................................................................................................................6 2. REVIEW OF EU AND UN-ECE REGULATIONS.....................................................................7 2.1 Cars and light duty vehicles ..........................................................................................7 -
Application of the Nox Reaction Model for Development of Low-Nox Combustion Technology for Pulverized Coals by Using the Gas Phase Stoichiometric Ratio Index
Energies 2011, 4, 545-562; doi:10.3390/en4030545 OPEN ACCESS energies ISSN 1996-1073 www.mdpi.com/journal/energies Article Application of the NOx Reaction Model for Development of Low-NOx Combustion Technology for Pulverized Coals by Using the Gas Phase Stoichiometric Ratio Index Masayuki Taniguchi *, Yuki Kamikawa, Tsuyoshi Shibata, Kenji Yamamoto and Hironobu Kobayashi Energy and Environmental Systems Laboratory, Hitachi, Ltd. Power Systems Company, 7-2-1 Omika-cho, Hitachi-shi, Ibaraki-ken, 319-1292, Japan; E-Mails: [email protected] (Y.K.); [email protected] (T.S.); [email protected] (K.Y.); [email protected] (H.K.) * Author to whom correspondence should be addressed; E-Mail: [email protected]; Tel.: +81-29-276-5889. Received: 9 December 2010; in revised form: 14 February 2011 / Accepted: 16 March 2011 / Published: 23 March 2011 Abstract: We previously proposed the gas phase stoichiometric ratio (SRgas) as an index to evaluate NOx concentration in fuel-rich flames. The SRgas index was defined as the amount of fuel required for stoichiometric combustion/amount of gasified fuel, where the amount of gasified fuel was the amount of fuel which had been released to the gas phase by pyrolysis, oxidation and gasification reactions. In the present study we found that SRgas was a good index to consider the gas phase reaction mechanism in fuel-rich pulverized coal flames. When SRgas < 1.0, NOx concentration was strongly influenced by the SRgas value. NOx concentration was also calculated by using a reaction model. The model was verified for various coals, particle diameters, reaction times, and initial oxygen concentrations. -
Ozone: Good up High, Bad Nearby
actions you can take High-Altitude “Good” Ozone Ground-Level “Bad” Ozone •Protect yourself against sunburn. When the UV Index is •Check the air quality forecast in your area. At times when the Air “high” or “very high”: Limit outdoor activities between 10 Quality Index (AQI) is forecast to be unhealthy, limit physical exertion am and 4 pm, when the sun is most intense. Twenty minutes outdoors. In many places, ozone peaks in mid-afternoon to early before going outside, liberally apply a broad-spectrum evening. Change the time of day of strenuous outdoor activity to avoid sunscreen with a Sun Protection Factor (SPF) of at least 15. these hours, or reduce the intensity of the activity. For AQI forecasts, Reapply every two hours or after swimming or sweating. For check your local media reports or visit: www.epa.gov/airnow UV Index forecasts, check local media reports or visit: www.epa.gov/sunwise/uvindex.html •Help your local electric utilities reduce ozone air pollution by conserving energy at home and the office. Consider setting your •Use approved refrigerants in air conditioning and thermostat a little higher in the summer. Participate in your local refrigeration equipment. Make sure technicians that work on utilities’ load-sharing and energy conservation programs. your car or home air conditioners or refrigerator are certified to recover the refrigerant. Repair leaky air conditioning units •Reduce air pollution from cars, trucks, gas-powered lawn and garden before refilling them. equipment, boats and other engines by keeping equipment properly tuned and maintained. During the summer, fill your gas tank during the cooler evening hours and be careful not to spill gasoline. -
Toxicological Profile for Acetone Draft for Public Comment
ACETONE 1 Toxicological Profile for Acetone Draft for Public Comment July 2021 ***DRAFT FOR PUBLIC COMMENT*** ACETONE ii DISCLAIMER Use of trade names is for identification only and does not imply endorsement by the Agency for Toxic Substances and Disease Registry, the Public Health Service, or the U.S. Department of Health and Human Services. This information is distributed solely for the purpose of pre dissemination public comment under applicable information quality guidelines. It has not been formally disseminated by the Agency for Toxic Substances and Disease Registry. It does not represent and should not be construed to represent any agency determination or policy. ***DRAFT FOR PUBLIC COMMENT*** ACETONE iii FOREWORD This toxicological profile is prepared in accordance with guidelines developed by the Agency for Toxic Substances and Disease Registry (ATSDR) and the Environmental Protection Agency (EPA). The original guidelines were published in the Federal Register on April 17, 1987. Each profile will be revised and republished as necessary. The ATSDR toxicological profile succinctly characterizes the toxicologic and adverse health effects information for these toxic substances described therein. Each peer-reviewed profile identifies and reviews the key literature that describes a substance's toxicologic properties. Other pertinent literature is also presented, but is described in less detail than the key studies. The profile is not intended to be an exhaustive document; however, more comprehensive sources of specialty information are referenced. The focus of the profiles is on health and toxicologic information; therefore, each toxicological profile begins with a relevance to public health discussion which would allow a public health professional to make a real-time determination of whether the presence of a particular substance in the environment poses a potential threat to human health. -
Focus on Sulfur Dioxide (SO2)
The information presented here reflects EPA's modeling of the Clear Skies Act of 2002. The Agency is in the process of updating this information to reflect modifications included in the Clear Skies Act of 2003. The revised information will be posted on the Agency's Clear Skies Web site (www.epa.gov/clearskies) as soon as possible. Overview of the Human Health and Environmental Effects of Power Generation: Focus on Sulfur Dioxide (SO2), Nitrogen Oxides (NOX) and Mercury (Hg) June 2002 Contents The Clear Skies Initiative is intended to reduce the health and environmental impacts of power generation. This document briefly summarizes the health and environmental effects of power generation, specifically those associated with sulfur dioxide (SO2), nitrogen oxides (NOX), and mercury (Hg). This document does not address the specific impacts of the Clear Skies Initiative. • Overview -- Emissions and transport • Fine Particles • Ozone (Smog) • Visibility • Acid Rain • Nitrogen Deposition • Mercury Photo Credits: 1: USDA; EPA; EPA; VTWeb.com 2: NADP 6: EPA 7: ?? 10: EPA 2 Electric Power Generation: Major Source of Emissions 2000 Sulfur Dioxide 2000 Nitrogen Oxides 1999 Mercury Fuel Combustion- Industrial Processing electric utilities Transportation Other stationary combustion * Miscellaneous * Other stationary combustion includes residential and commercial sources. • Power generation continues to be an important source of these major pollutants. • Power generation contributes 63% of SO2, 22% of NOX, and 37% of man-made mercury to the environment. 3 Overview of SO2, NOX, and Mercury Emissions, Transport, and Transformation • When emitted into the atmosphere, sulfur dioxide, nitrogen oxides, and mercury undergo chemical reactions to form compounds that can travel long distances. -
Method TO-11A
EPA/625/R-96/010b Compendium of Methods for the Determination of Toxic Organic Compounds in Ambient Air Second Edition Compendium Method TO-11A Determination of Formaldehyde in Ambient Air Using Adsorbent Cartridge Followed by High Performance Liquid Chromatography (HPLC) [Active Sampling Methodology] Center for Environmental Research Information Office of Research and Development U.S. Environmental Protection Agency Cincinnati, OH 45268 January 1999 Method TO-11A Acknowledgements This Method was prepared for publication in the Compendium of Methods for the Determination of Toxic Organic Compounds in Ambient Air, Second Edition (EPA/625/R-96/010b), which was prepared under Contract No. 68-C3-0315, WA No. 3-10, by Midwest Research Institute (MRI), as a subcontractor to Eastern Research Group, Inc. (ERG), and under the sponsorship of the U.S. Environmental Protection Agency (EPA). Justice A. Manning, John O. Burckle, and Scott Hedges, Center for Environmental Research Information (CERI), and Frank F. McElroy, National Exposure Research Laboratory (NERL), all in the EPA Office of Research and Development, were responsible for overseeing the preparation of this method. Additional support was provided by other members of the Compendia Workgroup, which include: • John O. Burckle, U.S. EPA, ORD, Cincinnati, OH • James L. Cheney, Corps of Engineers, Omaha, NB • Michael Davis, U.S. EPA, Region 7, KC, KS • Joseph B. Elkins Jr., U.S. EPA, OAQPS, RTP, NC • Robert G. Lewis, U.S. EPA, NERL, RTP, NC • Justice A. Manning, U.S. EPA, ORD, Cincinnati, OH • William A. McClenny, U.S. EPA, NERL, RTP, NC • Frank F. McElroy, U.S. EPA, NERL, RTP, NC • Heidi Schultz, ERG, Lexington, MA • William T. -
Effects of Mid-Level Ethanol Blends on Conventional Vehicle Emissions
NREL/CP-540-46570. Posted with permission. Presented at the 2009 SAE Powertrain, Fuels, and Lubricants Meeting, 2-4 November 2009, San Antonio, Texas 2009-01-2723 Effects of Mid-Level Ethanol Blends on Conventional Vehicle Emissions Keith Knoll National Renewable Energy Laboratory Brian West, Shean Huff, John Thomas Oak Ridge National Laboratory John Orban, Cynthia Cooper Battelle Memorial Institute ABSTRACT reductions in NMHC and CO were observed, as was a statistically significant increase in NOX emissions. Tests were conducted during 2008 on 16 late-model, Effects of ethanol on NMOG and NMHC emissions were conventional vehicles (1999 through 2007) to determine found to also be influenced by power-to-weight ratio, short-term effects of mid-level ethanol blends on while the effects on NOX emissions were found to be performance and emissions. Vehicle odometer readings influenced by engine displacement. ranged from 10,000 to 100,000 miles, and all vehicles conformed to federal emissions requirements for their federal certification level. The LA92 drive cycle, also INTRODUCTION known as the Unified Cycle, was used for testing as it was considered to more accurately represent real-world The United States’ Energy Independence and Security acceleration rates and speeds than the Federal Test Act (EISA) of 2007 calls on the nation to significantly Procedure (FTP) used for emissions certification testing. increase its use of renewable fuels to meet its Test fuels were splash-blends of up to 20 volume transportation energy needs.1 The law establishes a new percent ethanol with federal certification gasoline. Both renewable fuel standard (RFS) that requires 36 billion regulated and unregulated air-toxic emissions were gallons of renewable fuel to be used in the on-road measured.