UC Riverside Electronic Theses and Dissertations
Total Page:16
File Type:pdf, Size:1020Kb
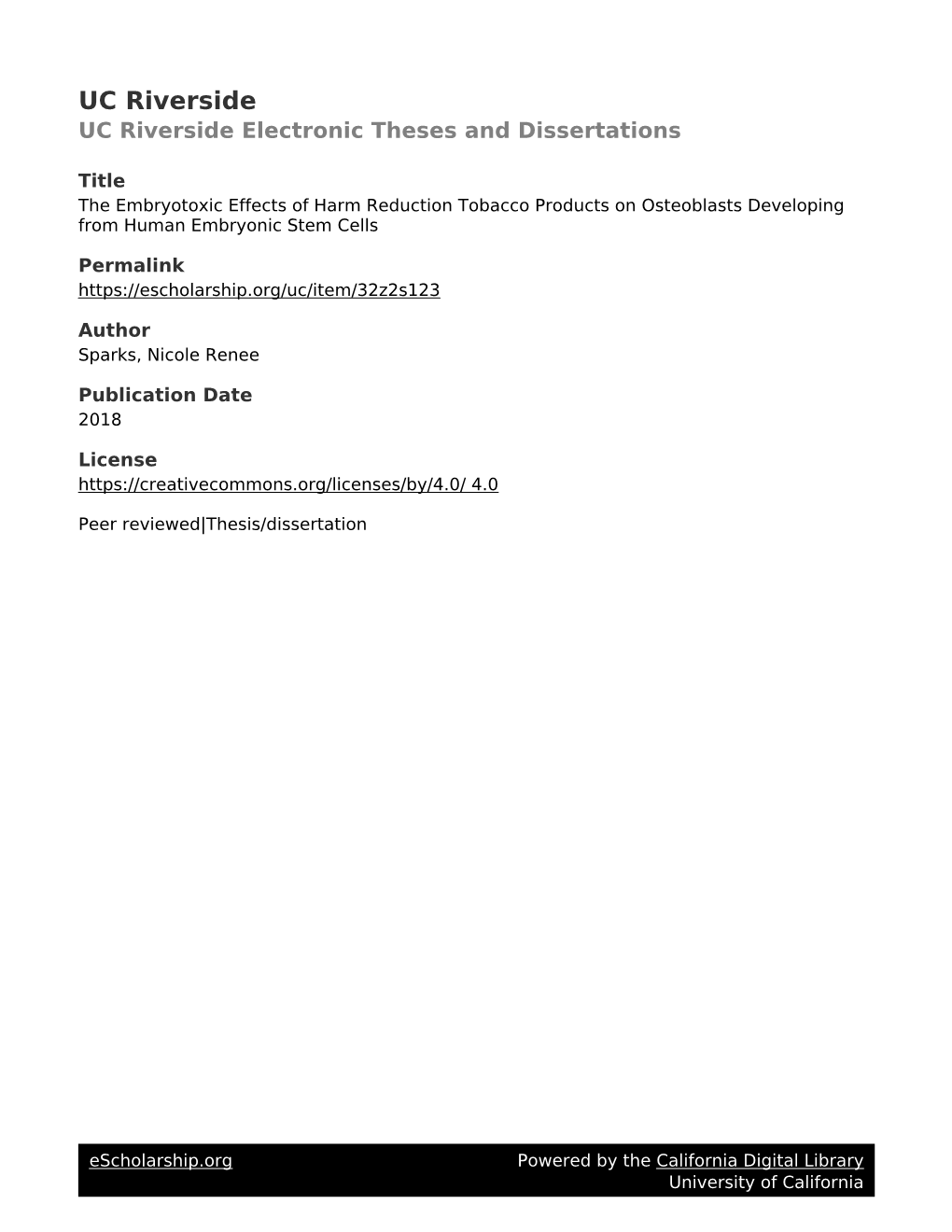
Load more
Recommended publications
-
Datasheet Blank Template
SAN TA C RUZ BI OTEC HNOL OG Y, INC . DIP2A (L-16): sc-67555 BACKGROUND APPLICATIONS DIP2A (Disco-interacting protein 2 homolog A), also known as DIP2, is a 1,571 DIP2A (L-16) is recommended for detection of DIP2A of human origin by amino acid nuclear protein. It is one of three human homologs (DIP2A, DIP2B Western Blotting (starting dilution 1:200, dilution range 1:100-1:1000), and DIP2C) of the Drosophila dip2 (disconnected-interacting protein 2) protein. immunoprecipitation [1-2 µg per 100-500 µg of total protein (1 ml of cell In Drosophila , dip2 interacts with disco, a protein required for neuronal con - lysate)], immunofluorescence (starting dilution 1:50, dilution range 1:50- nections in the visual systems of larvae and adults. The closest vertebrate 1:500) and solid phase ELISA (starting dilution 1:30, dilution range 1:30- homologs to disco are the basonuclin genes. In mice, DIP2 homologs show 1:3000). restricted expression to the brain. This suggests that, similar to the function DIP2A (L-16) is also recommended for detection of DIP2A, also designated of Drosphila dip2, vertebrate DIP2 homologs may play a role in the develop - Disco-interacting protein 2 homolog A, in additional species, including ment of the nervous system. Expressed ubiquitously with highest expression canine. in the brain, DIP2A is thought to function in signaling throughout the central nervous system by providing positional clues for axon patterning and pathfind - Suitable for use as control antibody for DIP2A siRNA (h): sc-62212, DIP2A ing . Four isoforms of DIP2A exist due to alternative splicing events. -
Download (PDF)
ANALYTICAL SCIENCES NOVEMBER 2020, VOL. 36 1 2020 © The Japan Society for Analytical Chemistry Supporting Information Fig. S1 Detailed MS/MS data of myoglobin. 17 2 ANALYTICAL SCIENCES NOVEMBER 2020, VOL. 36 Table S1 : The protein names (antigens) identified by pH 2.0 solution in the eluted-fraction. These proteins were identified one or more out of six analyses. Accession Description P08908 5-hydroxytryptamine receptor 1A OS=Homo sapiens GN=HTR1A PE=1 SV=3 - [5HT1A_HUMAN] Q9NRR6 72 kDa inositol polyphosphate 5-phosphatase OS=Homo sapiens GN=INPP5E PE=1 SV=2 - [INP5E_HUMAN] P82987 ADAMTS-like protein 3 OS=Homo sapiens GN=ADAMTSL3 PE=2 SV=4 - [ATL3_HUMAN] Q9Y6K8 Adenylate kinase isoenzyme 5 OS=Homo sapiens GN=AK5 PE=1 SV=2 - [KAD5_HUMAN] P02763 Alpha-1-acid glycoprotein 1 OS=Homo sapiens GN=ORM1 PE=1 SV=1 - [A1AG1_HUMAN] P19652 Alpha-1-acid glycoprotein 2 OS=Homo sapiens GN=ORM2 PE=1 SV=2 - [A1AG2_HUMAN] P01011 Alpha-1-antichymotrypsin OS=Homo sapiens GN=SERPINA3 PE=1 SV=2 - [AACT_HUMAN] P01009 Alpha-1-antitrypsin OS=Homo sapiens GN=SERPINA1 PE=1 SV=3 - [A1AT_HUMAN] P04217 Alpha-1B-glycoprotein OS=Homo sapiens GN=A1BG PE=1 SV=4 - [A1BG_HUMAN] P08697 Alpha-2-antiplasmin OS=Homo sapiens GN=SERPINF2 PE=1 SV=3 - [A2AP_HUMAN] P02765 Alpha-2-HS-glycoprotein OS=Homo sapiens GN=AHSG PE=1 SV=1 - [FETUA_HUMAN] P01023 Alpha-2-macroglobulin OS=Homo sapiens GN=A2M PE=1 SV=3 - [A2MG_HUMAN] P01019 Angiotensinogen OS=Homo sapiens GN=AGT PE=1 SV=1 - [ANGT_HUMAN] Q9NQ90 Anoctamin-2 OS=Homo sapiens GN=ANO2 PE=1 SV=2 - [ANO2_HUMAN] P01008 Antithrombin-III -
A Computational Approach for Defining a Signature of Β-Cell Golgi Stress in Diabetes Mellitus
Page 1 of 781 Diabetes A Computational Approach for Defining a Signature of β-Cell Golgi Stress in Diabetes Mellitus Robert N. Bone1,6,7, Olufunmilola Oyebamiji2, Sayali Talware2, Sharmila Selvaraj2, Preethi Krishnan3,6, Farooq Syed1,6,7, Huanmei Wu2, Carmella Evans-Molina 1,3,4,5,6,7,8* Departments of 1Pediatrics, 3Medicine, 4Anatomy, Cell Biology & Physiology, 5Biochemistry & Molecular Biology, the 6Center for Diabetes & Metabolic Diseases, and the 7Herman B. Wells Center for Pediatric Research, Indiana University School of Medicine, Indianapolis, IN 46202; 2Department of BioHealth Informatics, Indiana University-Purdue University Indianapolis, Indianapolis, IN, 46202; 8Roudebush VA Medical Center, Indianapolis, IN 46202. *Corresponding Author(s): Carmella Evans-Molina, MD, PhD ([email protected]) Indiana University School of Medicine, 635 Barnhill Drive, MS 2031A, Indianapolis, IN 46202, Telephone: (317) 274-4145, Fax (317) 274-4107 Running Title: Golgi Stress Response in Diabetes Word Count: 4358 Number of Figures: 6 Keywords: Golgi apparatus stress, Islets, β cell, Type 1 diabetes, Type 2 diabetes 1 Diabetes Publish Ahead of Print, published online August 20, 2020 Diabetes Page 2 of 781 ABSTRACT The Golgi apparatus (GA) is an important site of insulin processing and granule maturation, but whether GA organelle dysfunction and GA stress are present in the diabetic β-cell has not been tested. We utilized an informatics-based approach to develop a transcriptional signature of β-cell GA stress using existing RNA sequencing and microarray datasets generated using human islets from donors with diabetes and islets where type 1(T1D) and type 2 diabetes (T2D) had been modeled ex vivo. To narrow our results to GA-specific genes, we applied a filter set of 1,030 genes accepted as GA associated. -
Loss of DIP2C in RKO Cells Stimulates Changes in DNA
Larsson et al. BMC Cancer (2017) 17:487 DOI 10.1186/s12885-017-3472-5 RESEARCH ARTICLE Open Access Loss of DIP2C in RKO cells stimulates changes in DNA methylation and epithelial- mesenchymal transition Chatarina Larsson1, Muhammad Akhtar Ali1,2, Tatjana Pandzic1, Anders M. Lindroth3, Liqun He1,4 and Tobias Sjöblom1* Abstract Background: The disco-interacting protein 2 homolog C (DIP2C) gene is an uncharacterized gene found mutated in a subset of breast and lung cancers. To understand the role of DIP2C in tumour development we studied the gene in human cancer cells. Methods: We engineered human DIP2C knockout cells by genome editing in cancer cells. The growth properties of the engineered cells were characterised and transcriptome and methylation analyses were carried out to identify pathways deregulated by inactivation of DIP2C. Effects on cell death pathways and epithelial-mesenchymal transition traits were studied based on the results from expression profiling. Results: Knockout of DIP2C in RKO cells resulted in cell enlargement and growth retardation. Expression profiling revealed 780 genes for which the expression level was affected by the loss of DIP2C, including the tumour-suppressor encoding CDKN2A gene, the epithelial-mesenchymal transition (EMT) regulator-encoding ZEB1,andCD44 and CD24 that encode breast cancer stem cell markers. Analysis of DNA methylation showed more than 30,000 sites affected by differential methylation, the majority of which were hypomethylated following loss of DIP2C. Changes in DNA methylation at promoter regions were strongly correlated to changes in gene expression, and genes involved with EMT and cell death were enriched among the differentially regulated genes. -
WES Gene Package Multiple Congenital Anomalie.Xlsx
Whole Exome Sequencing Gene package Multiple congenital anomalie, version 5, 1‐2‐2018 Technical information DNA was enriched using Agilent SureSelect Clinical Research Exome V2 capture and paired‐end sequenced on the Illumina platform (outsourced). The aim is to obtain 8.1 Giga base pairs per exome with a mapped fraction of 0.99. The average coverage of the exome is ~50x. Duplicate reads are excluded. Data are demultiplexed with bcl2fastq Conversion Software from Illumina. Reads are mapped to the genome using the BWA‐MEM algorithm (reference: http://bio‐bwa.sourceforge.net/). Variant detection is performed by the Genome Analysis Toolkit HaplotypeCaller (reference: http://www.broadinstitute.org/gatk/). The detected variants are filtered and annotated with Cartagenia software and classified with Alamut Visual. It is not excluded that pathogenic mutations are being missed using this technology. At this moment, there is not enough information about the sensitivity of this technique with respect to the detection of deletions and duplications of more than 5 nucleotides and of somatic mosaic mutations (all types of sequence changes). HGNC approved Phenotype description including OMIM phenotype ID(s) OMIM median depth % covered % covered % covered gene symbol gene ID >10x >20x >30x A4GALT [Blood group, P1Pk system, P(2) phenotype], 111400 607922 101 100 100 99 [Blood group, P1Pk system, p phenotype], 111400 NOR polyagglutination syndrome, 111400 AAAS Achalasia‐addisonianism‐alacrimia syndrome, 231550 605378 73 100 100 100 AAGAB Keratoderma, palmoplantar, -
2016 Yet Idil 1167460 Ethesis
This electronic thesis or dissertation has been downloaded from the King’s Research Portal at https://kclpure.kcl.ac.uk/portal/ INTEGRATED EPIGENOMICS AND METABOLOMICS ANALYSIS IN TWINS Yet, Idil Awarding institution: King's College London The copyright of this thesis rests with the author and no quotation from it or information derived from it may be published without proper acknowledgement. END USER LICENCE AGREEMENT Unless another licence is stated on the immediately following page this work is licensed under a Creative Commons Attribution-NonCommercial-NoDerivatives 4.0 International licence. https://creativecommons.org/licenses/by-nc-nd/4.0/ You are free to copy, distribute and transmit the work Under the following conditions: Attribution: You must attribute the work in the manner specified by the author (but not in any way that suggests that they endorse you or your use of the work). Non Commercial: You may not use this work for commercial purposes. No Derivative Works - You may not alter, transform, or build upon this work. Any of these conditions can be waived if you receive permission from the author. Your fair dealings and other rights are in no way affected by the above. Take down policy If you believe that this document breaches copyright please contact [email protected] providing details, and we will remove access to the work immediately and investigate your claim. Download date: 01. Oct. 2021 INTEGRATED EPIGENOMICS AND METABOLOMICS ANALYSIS IN TWINS IDIL YET KING’S COLLEGE LONDON FACULTY OF LIFE SCIENCES & MEDICINE DIVISION OF GENETICS & MOLECULAR MEDICINE DEPARTMENT OF TWIN RESEARCH AND GENETIC EPIDEMIOLOGY Submitted in particular fulfilment of the requirements for the degree of Doctor of Philosophy 2016 1 ABSTRACT Epigenetics and metabolomics are rapidly growing areas of research, in part due to recent advances in technology that have allowed for a wide coverage of the human genome. -
1 Mutational Heterogeneity in Cancer Akash Kumar a Dissertation
Mutational Heterogeneity in Cancer Akash Kumar A dissertation Submitted in partial fulfillment of requirements for the degree of Doctor of Philosophy University of Washington 2014 June 5 Reading Committee: Jay Shendure Pete Nelson Mary Claire King Program Authorized to Offer Degree: Genome Sciences 1 University of Washington ABSTRACT Mutational Heterogeneity in Cancer Akash Kumar Chair of the Supervisory Committee: Associate Professor Jay Shendure Department of Genome Sciences Somatic mutation plays a key role in the formation and progression of cancer. Differences in mutation patterns likely explain much of the heterogeneity seen in prognosis and treatment response among patients. Recent advances in massively parallel sequencing have greatly expanded our capability to investigate somatic mutation. Genomic profiling of tumor biopsies could guide the administration of targeted therapeutics on the basis of the tumor’s collection of mutations. Central to the success of this approach is the general applicability of targeted therapies to a patient’s entire tumor burden. This requires a better understanding of the genomic heterogeneity present both within individual tumors (intratumoral) and amongst tumors from the same patient (intrapatient). My dissertation is broadly organized around investigating mutational heterogeneity in cancer. Three projects are discussed in detail: analysis of (1) interpatient and (2) intrapatient heterogeneity in men with disseminated prostate cancer, and (3) investigation of regional intratumoral heterogeneity in -
Supplementary Materials
Supplementary materials Supplementary Table S1: MGNC compound library Ingredien Molecule Caco- Mol ID MW AlogP OB (%) BBB DL FASA- HL t Name Name 2 shengdi MOL012254 campesterol 400.8 7.63 37.58 1.34 0.98 0.7 0.21 20.2 shengdi MOL000519 coniferin 314.4 3.16 31.11 0.42 -0.2 0.3 0.27 74.6 beta- shengdi MOL000359 414.8 8.08 36.91 1.32 0.99 0.8 0.23 20.2 sitosterol pachymic shengdi MOL000289 528.9 6.54 33.63 0.1 -0.6 0.8 0 9.27 acid Poricoic acid shengdi MOL000291 484.7 5.64 30.52 -0.08 -0.9 0.8 0 8.67 B Chrysanthem shengdi MOL004492 585 8.24 38.72 0.51 -1 0.6 0.3 17.5 axanthin 20- shengdi MOL011455 Hexadecano 418.6 1.91 32.7 -0.24 -0.4 0.7 0.29 104 ylingenol huanglian MOL001454 berberine 336.4 3.45 36.86 1.24 0.57 0.8 0.19 6.57 huanglian MOL013352 Obacunone 454.6 2.68 43.29 0.01 -0.4 0.8 0.31 -13 huanglian MOL002894 berberrubine 322.4 3.2 35.74 1.07 0.17 0.7 0.24 6.46 huanglian MOL002897 epiberberine 336.4 3.45 43.09 1.17 0.4 0.8 0.19 6.1 huanglian MOL002903 (R)-Canadine 339.4 3.4 55.37 1.04 0.57 0.8 0.2 6.41 huanglian MOL002904 Berlambine 351.4 2.49 36.68 0.97 0.17 0.8 0.28 7.33 Corchorosid huanglian MOL002907 404.6 1.34 105 -0.91 -1.3 0.8 0.29 6.68 e A_qt Magnogrand huanglian MOL000622 266.4 1.18 63.71 0.02 -0.2 0.2 0.3 3.17 iolide huanglian MOL000762 Palmidin A 510.5 4.52 35.36 -0.38 -1.5 0.7 0.39 33.2 huanglian MOL000785 palmatine 352.4 3.65 64.6 1.33 0.37 0.7 0.13 2.25 huanglian MOL000098 quercetin 302.3 1.5 46.43 0.05 -0.8 0.3 0.38 14.4 huanglian MOL001458 coptisine 320.3 3.25 30.67 1.21 0.32 0.9 0.26 9.33 huanglian MOL002668 Worenine -
Whole-Exome Sequencing of Metastatic Cancer and Biomarkers of Treatment Response
Supplementary Online Content Beltran H, Eng K, Mosquera JM, et al. Whole-exome sequencing of metastatic cancer and biomarkers of treatment response. JAMA Oncol. Published online May 28, 2015. doi:10.1001/jamaoncol.2015.1313 eMethods eFigure 1. A schematic of the IPM Computational Pipeline eFigure 2. Tumor purity analysis eFigure 3. Tumor purity estimates from Pathology team versus computationally (CLONET) estimated tumor purities values for frozen tumor specimens (Spearman correlation 0.2765327, p- value = 0.03561) eFigure 4. Sequencing metrics Fresh/frozen vs. FFPE tissue eFigure 5. Somatic copy number alteration profiles by tumor type at cytogenetic map location resolution; for each cytogenetic map location the mean genes aberration frequency is reported eFigure 6. The 20 most frequently aberrant genes with respect to copy number gains/losses detected per tumor type eFigure 7. Top 50 genes with focal and large scale copy number gains (A) and losses (B) across the cohort eFigure 8. Summary of total number of copy number alterations across PM tumors eFigure 9. An example of tumor evolution looking at serial biopsies from PM222, a patient with metastatic bladder carcinoma eFigure 10. PM12 somatic mutations by coverage and allele frequency (A) and (B) mutation correlation between primary (y- axis) and brain metastasis (x-axis) eFigure 11. Point mutations across 5 metastatic sites of a 55 year old patient with metastatic prostate cancer at time of rapid autopsy eFigure 12. CT scans from patient PM137, a patient with recurrent platinum refractory metastatic urothelial carcinoma eFigure 13. Tracking tumor genomics between primary and metastatic samples from patient PM12 eFigure 14. -
Wo2017/132291
(12) INTERNATIONAL APPLICATION PUBLISHED UNDER THE PATENT COOPERATION TREATY (PCT) (19) World Intellectual Property Organization International Bureau (10) International Publication Number (43) International Publication Date W O 2017/132291 A l 3 August 2017 (03.08.2017) P O P C T (51) International Patent Classification: [US/US]; 77 Massachusetts Avenue, Cambridge, MA A61K 48/00 (2006.01) C12Q 1/68 (2006.01) 02139 (US). THE GENERAL HOSPITAL CORPORA¬ A61K 39/395 (2006.01) G01N 33/574 (2006.01) TION [US/US]; 55 Fruit Street, Boston, MA 021 14 (US). C12N 15/11 (2006.01) (72) Inventors; and (21) International Application Number: (71) Applicants : REGEV, Aviv [US/US]; 415 Main Street, PCT/US2017/014995 Cambridge, MA 02142 (US). BERNSTEIN, Bradley [US/US]; 55 Fruit Street, Boston, MA 021 14 (US). (22) International Filing Date: TIROSH, Itay [US/US]; 415 Main Street, Cambridge, 25 January 20 17 (25.01 .2017) MA 02142 (US). SUVA, Mario [US/US]; 55 Fruit Street, (25) Filing Language: English Bostn, MA 02144 (US). ROZENBALTT-ROSEN, Orit [US/US]; 415 Main Street, Cambridge, MA 02142 (US). (26) Publication Language: English (74) Agent: NIX, F., Brent; Johnson, Marcou & Isaacs, LLC, (30) Priority Data: 317A East Liberty St., Savannah, GA 31401 (US). 62/286,850 25 January 2016 (25.01.2016) US 62/437,558 2 1 December 201 6 (21. 12.2016) US (81) Designated States (unless otherwise indicated, for every kind of national protection available): AE, AG, AL, AM, (71) Applicants: THE BROAD INSTITUTE, INC. [US/US]; AO, AT, AU, AZ, BA, BB, BG, BH, BN, BR, BW, BY, 415 Main Street, Cambridge, MA 02142 (US). -
Open Full Page
Published OnlineFirst February 10, 2017; DOI: 10.1158/2159-8290.CD-16-1045 RESEARCH ARTICLE Interaction Landscape of Inherited Polymorphisms with Somatic Events in Cancer Hannah Carter 1 , 2 , 3 , 4 , Rachel Marty 5 , Matan Hofree 6 , Andrew M. Gross 5 , James Jensen 5 , Kathleen M. Fisch1,2,3,7 , Xingyu Wu 2 , Christopher DeBoever 5 , Eric L. Van Nostrand 4,8 , Yan Song 4,8 , Emily Wheeler 4,8 , Jason F. Kreisberg 1,3 , Scott M. Lippman 2 , Gene W. Yeo 4,8 , J. Silvio Gutkind 2 , 3 , and Trey Ideker 1 , 2 , 3 , 4 , 5,6 Downloaded from cancerdiscovery.aacrjournals.org on September 27, 2021. © 2017 American Association for Cancer Research. Published OnlineFirst February 10, 2017; DOI: 10.1158/2159-8290.CD-16-1045 ABSTRACT Recent studies have characterized the extensive somatic alterations that arise dur- ing cancer. However, the somatic evolution of a tumor may be signifi cantly affected by inherited polymorphisms carried in the germline. Here, we analyze genomic data for 5,954 tumors to reveal and systematically validate 412 genetic interactions between germline polymorphisms and major somatic events, including tumor formation in specifi c tissues and alteration of specifi c cancer genes. Among germline–somatic interactions, we found germline variants in RBFOX1 that increased incidence of SF3B1 somatic mutation by 8-fold via functional alterations in RNA splicing. Similarly, 19p13.3 variants were associated with a 4-fold increased likelihood of somatic mutations in PTEN. In support of this associ- ation, we found that PTEN knockdown sensitizes the MTOR pathway to high expression of the 19p13.3 gene GNA11 . -
Table SII. Significantly Differentially Expressed Mrnas of GSE23558 Data Series with the Criteria of Adjusted P<0.05 And
Table SII. Significantly differentially expressed mRNAs of GSE23558 data series with the criteria of adjusted P<0.05 and logFC>1.5. Probe ID Adjusted P-value logFC Gene symbol Gene title A_23_P157793 1.52x10-5 6.91 CA9 carbonic anhydrase 9 A_23_P161698 1.14x10-4 5.86 MMP3 matrix metallopeptidase 3 A_23_P25150 1.49x10-9 5.67 HOXC9 homeobox C9 A_23_P13094 3.26x10-4 5.56 MMP10 matrix metallopeptidase 10 A_23_P48570 2.36x10-5 5.48 DHRS2 dehydrogenase A_23_P125278 3.03x10-3 5.40 CXCL11 C-X-C motif chemokine ligand 11 A_23_P321501 1.63x10-5 5.38 DHRS2 dehydrogenase A_23_P431388 2.27x10-6 5.33 SPOCD1 SPOC domain containing 1 A_24_P20607 5.13x10-4 5.32 CXCL11 C-X-C motif chemokine ligand 11 A_24_P11061 3.70x10-3 5.30 CSAG1 chondrosarcoma associated gene 1 A_23_P87700 1.03x10-4 5.25 MFAP5 microfibrillar associated protein 5 A_23_P150979 1.81x10-2 5.25 MUCL1 mucin like 1 A_23_P1691 2.71x10-8 5.12 MMP1 matrix metallopeptidase 1 A_23_P350005 2.53x10-4 5.12 TRIML2 tripartite motif family like 2 A_24_P303091 1.23x10-3 4.99 CXCL10 C-X-C motif chemokine ligand 10 A_24_P923612 1.60x10-5 4.95 PTHLH parathyroid hormone like hormone A_23_P7313 6.03x10-5 4.94 SPP1 secreted phosphoprotein 1 A_23_P122924 2.45x10-8 4.93 INHBA inhibin A subunit A_32_P155460 6.56x10-3 4.91 PICSAR P38 inhibited cutaneous squamous cell carcinoma associated lincRNA A_24_P686965 8.75x10-7 4.82 SH2D5 SH2 domain containing 5 A_23_P105475 7.74x10-3 4.70 SLCO1B3 solute carrier organic anion transporter family member 1B3 A_24_P85099 4.82x10-5 4.67 HMGA2 high mobility group AT-hook 2 A_24_P101651