Diversity, Stability and Connectivity of Symbiodinium Populations at Various Spatial Scales
Total Page:16
File Type:pdf, Size:1020Kb
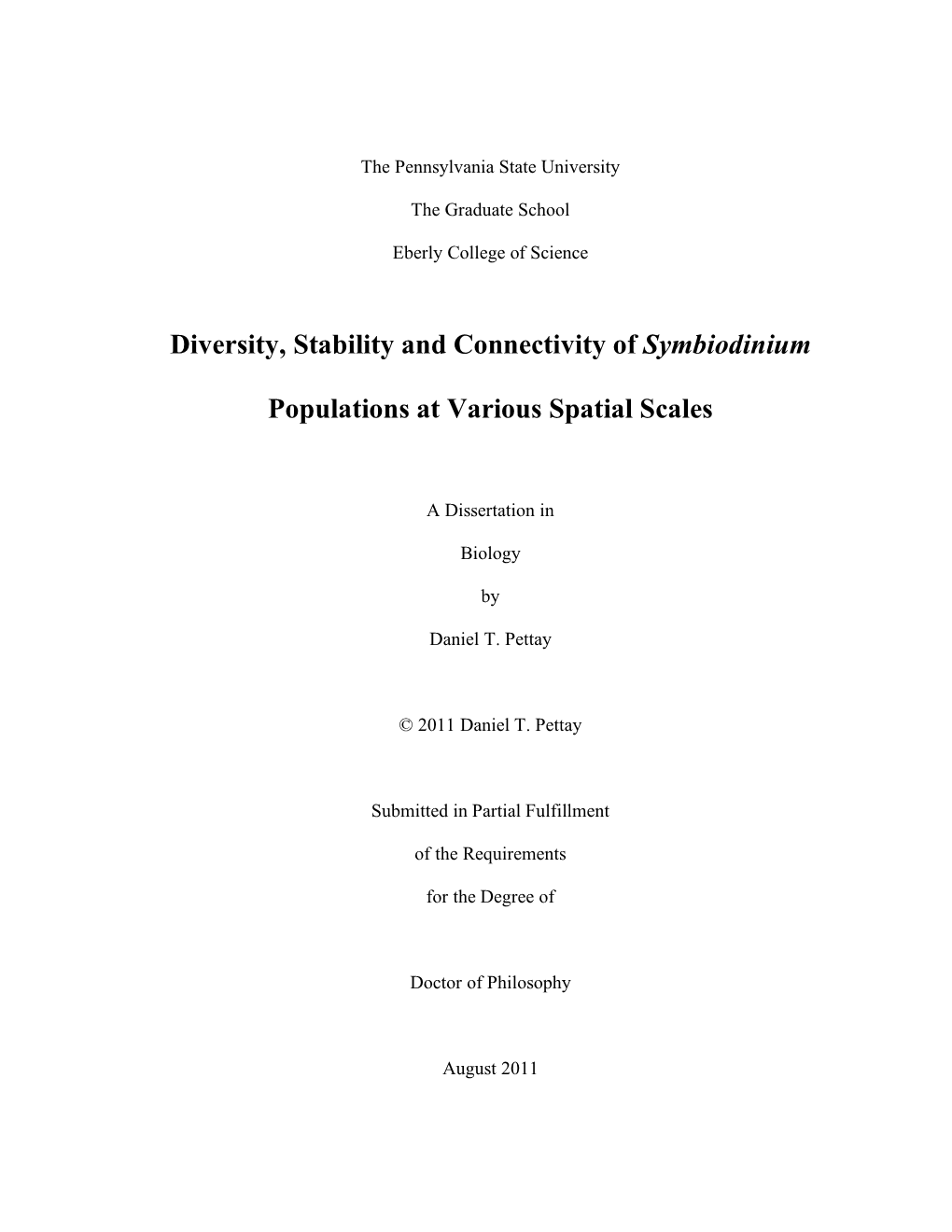
Load more
Recommended publications
-
Microsatellite Loci for Symbiodinium Goreaui and Other Clade C Symbiodinium
Conservation Genet Resour DOI 10.1007/s12686-013-0023-5 MICROSATELLITE LETTERS Microsatellite loci for Symbiodinium goreaui and other Clade C Symbiodinium Drew C. Wham • Margaux Carmichael • Todd C. LaJeunesse Received: 27 June 2013 / Accepted: 12 August 2013 Ó Springer Science+Business Media Dordrecht 2013 Abstract The genus Symbiodinium comprises a diverse of ‘‘Clades’’, with the most ecologically dominant and group of dinoflagellates known for their obligate relation- diverse being Clade C (LaJeunesse et al. 2004). While the ship with reef–building corals. Members of the sub-genus group includes phylogenetic types that display narrow and ‘clade C’ are abundant, geographically wide-spread, as broad thermal tolerances, members of clade C are often the well as genetically and ecologically diverse. Coral colonies most severely impacted during bleaching events. For this harboring clade C are often the most exposed to physical reason, their symbiosis biology, dispersal capabilities and stressors. The genotypic diversity, dispersal and genetic genetic identities are of increasing importance to conser- connectivity exhibited by these Symbiodinium are the vation efforts as climates change globally, further affecting subjects of an increasing number of population genetic reef coral communities. studies utilizing microsatellites. Here we describe 18 new Coral endosymbionts are increasingly being studied with microsatellite loci and test their utility across four common multi-locus techniques because this approach offers genetic clade C types. We obtained multi-locus genotypes with resolution to the level of the individual. High-resolution individual level resolution in each of these types. Our markers, however, are not yet available for most Symbiodi- results indicate that multi-locus genotypes can be obtained nium spp. -
Unfolding the Secrets of Coral–Algal Symbiosis
The ISME Journal (2015) 9, 844–856 & 2015 International Society for Microbial Ecology All rights reserved 1751-7362/15 www.nature.com/ismej ORIGINAL ARTICLE Unfolding the secrets of coral–algal symbiosis Nedeljka Rosic1, Edmund Yew Siang Ling2, Chon-Kit Kenneth Chan3, Hong Ching Lee4, Paulina Kaniewska1,5,DavidEdwards3,6,7,SophieDove1,8 and Ove Hoegh-Guldberg1,8,9 1School of Biological Sciences, The University of Queensland, St Lucia, Queensland, Australia; 2University of Queensland Centre for Clinical Research, The University of Queensland, Herston, Queensland, Australia; 3School of Agriculture and Food Sciences, The University of Queensland, St Lucia, Queensland, Australia; 4The Kinghorn Cancer Centre, Garvan Institute of Medical Research, Sydney, New South Wales, Australia; 5Australian Institute of Marine Science, Townsville, Queensland, Australia; 6School of Plant Biology, University of Western Australia, Perth, Western Australia, Australia; 7Australian Centre for Plant Functional Genomics, The University of Queensland, St Lucia, Queensland, Australia; 8ARC Centre of Excellence for Coral Reef Studies, The University of Queensland, St Lucia, Queensland, Australia and 9Global Change Institute and ARC Centre of Excellence for Coral Reef Studies, The University of Queensland, St Lucia, Queensland, Australia Dinoflagellates from the genus Symbiodinium form a mutualistic symbiotic relationship with reef- building corals. Here we applied massively parallel Illumina sequencing to assess genetic similarity and diversity among four phylogenetically diverse dinoflagellate clades (A, B, C and D) that are commonly associated with corals. We obtained more than 30 000 predicted genes for each Symbiodinium clade, with a majority of the aligned transcripts corresponding to sequence data sets of symbiotic dinoflagellates and o2% of sequences having bacterial or other foreign origin. -
(Symbiodinium) in Scleractinian Corals from Tropical Reefs in Southern Hainan
Journal of Systematics and Evolution 49 (6): 598–605 (2011) doi: 10.1111/j.1759-6831.2011.00161.x Research Article Low genetic diversity of symbiotic dinoflagellates (Symbiodinium) in scleractinian corals from tropical reefs in southern Hainan Island, China 1,2Guo-Wei ZHOU 1,2Hui HUANG∗ 1(Key Laboratory of Marine Bio-resources Sustainable Utilization, South China Sea Institute of Oceanology, Chinese Academy of Sciences, Guangzhou 510301, China) 2(Tropical Marine Biological Research Station in Hainan, Chinese Academy of Sciences, Sanya 572000, China) Abstract Endosymbiotic dinoflagellates in the genus Symbiodinium are among the most abundant and important group of photosynthetic protists found in coral reef ecosystems. In order to further characterize this diversity and compare with other regions of the Pacific, samples from 44 species of scleractinian corals representing 20 genera and 9 families, were collected from tropical reefs in southern Hainan Island, China. Denaturing gradient gel electrophoresis fingerprinting of the ribosomal internal transcribed spacer 2 identified 11 genetically distinct Symbiodinium types that have been reported previously. The majority of reef-building coral species (88.6%) harbored only one subcladal type of symbiont, dominated by host-generalist C1 and C3, and was influenced little by the host’s apparent mode of symbiont acquisition. Some species harbored more than one clade of Symbiodinium (clades C, D) concurrently. Although geographically isolated from the rest of the Pacific, the symbiont diversity in southern Hainan Island was relatively low and similar to both the Great Barrier Reef and Hawaii symbiont assemblages (dominated by clade C Symbiodinium). These results indicate that a specialist symbiont is not a prerequisite for existence in remote and isolated areas, but additional work in other geographic regions is necessary to test this idea. -
State of the Coral Triangle: Solomon Islands
State of the Coral Triangle: Solomon Islands One of a series of six reports on the status of marine resources in the western Pacific Ocean, the State of the Coral Triangle: Solomon Islands describes the biophysical characteristics of Solomon Islands’ coastal and marine ecosystems, the manner in which they are being exploited, the framework in place that governs their use, the socioeconomic characteristics of the communities that use them, and the environmental threats posed by the manner in which STATE OF THE CORAL TRIANGLE: they are being used. It explains the country’s national plan of action to address these threats and improve marine resource management. Solomon Islands About the Asian Development Bank ADB’s vision is an Asia and Pacific region free of poverty. Its mission is to help its developing member countries reduce poverty and improve the quality of life of their people. Despite the region’s many successes, it remains home to approximately two-thirds of the world’s poor: 1.6 billion people who live on less than $2 a day, with 733 million struggling on less than $1.25 a day. ADB is committed to reducing poverty through inclusive economic growth, environmentally sustainable growth, and regional integration. Based in Manila, ADB is owned by 67 members, including 48 from the region. Its main instruments for helping its developing member countries are policy dialogue, loans, equity investments, guarantees, grants, and technical assistance. Asian Development Bank 6 ADB Avenue, Mandaluyong City 1550 Metro Manila, Philippines www.adb.org Printed on recycled paper Printed in the Philippines STATE OF THE CORAL TRIANGLE: Solomon Islands © 2014 Asian Development Bank All rights reserved. -
Microbial Invasion of the Caribbean by an Indo-Pacific Coral Zooxanthella
Microbial invasion of the Caribbean by an Indo-Pacific coral zooxanthella D. Tye Pettaya,b,1, Drew C. Whama, Robin T. Smithc,d, Roberto Iglesias-Prietoc, and Todd C. LaJeunessea,e,1 aDepartment of Biology, The Pennsylvania State University, University Park, PA 16802; bCollege of Earth, Ocean, and Environment, University of Delaware, Lewes, DE 19958; cUnidad Académica de Sistemas Arrecifales (Puerto Morelos), Instituto de Ciencias del Mar y Limnología, Universidad Nacional Autónoma de México, CP 77500 Cancún, Mexico; dScience Under Sail Institute for Exploration, Sarasota, FL 34230; and ePenn State Institutes of Energy and the Environment, University Park, PA 16802 Edited by Nancy A. Moran, University of Texas at Austin, Austin, TX, and approved April 28, 2015 (received for review February 11, 2015) Human-induced environmental changes have ushered in the rapid coral communities distributed across broad geographic areas decline of coral reef ecosystems, particularly by disrupting the over the decadal ecological timescales that are necessary to keep symbioses between reef-building corals and their photosymbionts. pace with the current rate of warming. However, escalating stressful conditions enable some symbionts Research on the diversity and ecology of coral symbionts to thrive as opportunists. We present evidence that a stress-tolerant suggests that episodes of stressful warming may facilitate the “zooxanthella” from the Indo-Pacific Ocean, Symbiodinium trenchii, spread of ecologically rare or opportunistic species (13). The has rapidly spread to coral communities across the Greater Carib- severe mass bleaching and mortality of eastern Caribbean corals bean. In marked contrast to populations from the Indo-Pacific, in 2005 corresponded with an increased prevalence and abun- Atlantic populations of S. -
The AREA's RICHNESS
The AREA’S RICHNESS hile there is little doubt that the Coral Triangle is the richest marine environment on the planet, the reasons for the richness are hotly debated. Is it because Wthe richest taxonomic groups originated in the Coral Triangle and dispersed to the rest of the world? Or is it because of the overlap of flora and fauna from the West Pacific Ocean and Indian Ocean? As it turns out, the only thing that is clear is that the reasons for the area’s richness are complex and no single model explains it all. Some of the many factors that influence the diversity of the Coral Triangle are the geological history of the region, including plate tectonics and sea-level fluctuations; how species disperse and the factors that facilitate or inhibit dispersal; general biogeographic patterns and evolutionary forces. The tropics are generally more species rich than temperate and polar areas particularly because of the constant sunlight regime and weather stability. Without a winter period, organisms can flourish year round and put more energy into specialization than into preparing for long periods with reduced sunlight. This is as true in the marine realm as it is in the forests, where light and relatively constant warm water temperatures persist throughout the year. However, coastal tropical waters are relatively nutrient poor. The annual changes in water temperatures of the temperate and polar seas produce mixing when the surface waters cool and sink to the bottom. The displaced bottom waters, rich with dead plankton that has sank to the depths, are forced to the surface and result in Due to ocean circulation patterns and the rotation of the Earth, As the glaciers melted and sea levels rose, the newly evolved species huge explosions of zooplankton and fish populations. -
Symbiodinium Genomes Reveal Adaptive Evolution of Functions Related to Symbiosis
bioRxiv preprint doi: https://doi.org/10.1101/198762; this version posted October 5, 2017. The copyright holder for this preprint (which was not certified by peer review) is the author/funder, who has granted bioRxiv a license to display the preprint in perpetuity. It is made available under aCC-BY-NC-ND 4.0 International license. 1 Article 2 Symbiodinium genomes reveal adaptive evolution of 3 functions related to symbiosis 4 Huanle Liu1, Timothy G. Stephens1, Raúl A. González-Pech1, Victor H. Beltran2, Bruno 5 Lapeyre3,4, Pim Bongaerts5, Ira Cooke3, David G. Bourne2,6, Sylvain Forêt7,*, David J. 6 Miller3, Madeleine J. H. van Oppen2,8, Christian R. Voolstra9, Mark A. Ragan1 and Cheong 7 Xin Chan1,10,† 8 1Institute for Molecular Bioscience, The University of Queensland, Brisbane, QLD 4072, 9 Australia 10 2Australian Institute of Marine Science, Townsville, QLD 4810, Australia 11 3ARC Centre of Excellence for Coral Reef Studies and Department of Molecular and Cell 12 Biology, James Cook University, Townsville, QLD 4811, Australia 13 4Laboratoire d’excellence CORAIL, Centre de Recherches Insulaires et Observatoire de 14 l’Environnement, Moorea 98729, French Polynesia 15 5Global Change Institute, The University of Queensland, Brisbane, QLD 4072, Australia 16 6College of Science and Engineering, James Cook University, Townsville, QLD 4811, 17 Australia 18 7Research School of Biology, Australian National University, Canberra, ACT 2601, Australia 19 8School of BioSciences, The University of Melbourne, VIC 3010, Australia 1 bioRxiv preprint doi: https://doi.org/10.1101/198762; this version posted October 5, 2017. The copyright holder for this preprint (which was not certified by peer review) is the author/funder, who has granted bioRxiv a license to display the preprint in perpetuity. -
The Symbiotic Life of Symbiodinium in the Open Ocean Within a New Species of Calcifying Ciliate (Tiarina Sp.)
The ISME Journal (2016) 10, 1424–1436 © 2016 International Society for Microbial Ecology All rights reserved 1751-7362/16 www.nature.com/ismej ORIGINAL ARTICLE The symbiotic life of Symbiodinium in the open ocean within a new species of calcifying ciliate (Tiarina sp.) Solenn Mordret1,2,5, Sarah Romac1,2, Nicolas Henry1,2, Sébastien Colin1,2, Margaux Carmichael1,2, Cédric Berney1,2, Stéphane Audic1,2, Daniel J Richter1,2, Xavier Pochon3,4, Colomban de Vargas1,2 and Johan Decelle1,2,6 1EPEP—Evolution des Protistes et des Ecosystèmes Pélagiques—team, Sorbonne Universités, UPMC Univ Paris 06, UMR 7144, Station Biologique de Roscoff, Roscoff, France; 2CNRS, UMR 7144, Station Biologique de Roscoff, Roscoff, France; 3Coastal and Freshwater Group, Cawthron Institute, Nelson, New Zealand and 4Institute of Marine Science, University of Auckland, Auckland, New Zealand Symbiotic partnerships between heterotrophic hosts and intracellular microalgae are common in tropical and subtropical oligotrophic waters of benthic and pelagic marine habitats. The iconic example is the photosynthetic dinoflagellate genus Symbiodinium that establishes mutualistic symbioses with a wide diversity of benthic hosts, sustaining highly biodiverse reef ecosystems worldwide. Paradoxically, although various species of photosynthetic dinoflagellates are prevalent eukaryotic symbionts in pelagic waters, Symbiodinium has not yet been reported in symbiosis within oceanic plankton, despite its high propensity for the symbiotic lifestyle. Here we report a new pelagic photosymbiosis between a calcifying ciliate host and the microalga Symbiodinium in surface ocean waters. Confocal and scanning electron microscopy, together with an 18S rDNA-based phylogeny, showed that the host is a new ciliate species closely related to Tiarina fusus (Colepidae). -
0Ca27eedadadd00c93df6016e4
Long-Range Dispersal and High-Latitude Environments Influence the Population Structure of a “Stress-Tolerant” Dinoflagellate Endosymbiont D. Tye Pettay1,2*, Todd C. LaJeunesse1 1 Department of Biology, Pennsylvania State University, University Park, Pennsylvania, United States of America, 2 College of Earth, Ocean, and Environment, University of Delaware, Lewes, Delaware, United States of America Abstract The migration and dispersal of stress-tolerant symbiotic dinoflagellates (genus Symbiodinium) may influence the response of symbiotic reef-building corals to a warming climate. We analyzed the genetic structure of the stress- tolerant endosymbiont, Symbiodinium glynni nomen nudum (ITS2 - D1), obtained from Pocillopora colonies that dominate eastern Pacific coral communities. Eleven microsatellite loci identified genotypically diverse populations with minimal genetic subdivision throughout the Eastern Tropical Pacific, encompassing 1000’s of square kilometers from mainland Mexico to the Galapagos Islands. The lack of population differentiation over these distances corresponds with extensive regional host connectivity and indicates that Pocillopora larvae, which maternally inherit their symbionts, aid in the dispersal of this symbiont. In contrast to its host, however, subtropical populations of S. glynni in the Gulf of California (Sea of Cortez) were strongly differentiated from populations in tropical eastern Pacific. Selection pressures related to large seasonal fluctuations in temperature and irradiance likely explain this abrupt genetic -
Symbiodinium Genomes Reveal Adaptive Evolution of Functions Related to Coral-Dinoflagellate Symbiosis
Corrected: Publisher correction ARTICLE DOI: 10.1038/s42003-018-0098-3 OPEN Symbiodinium genomes reveal adaptive evolution of functions related to coral-dinoflagellate symbiosis Huanle Liu1, Timothy G. Stephens1, Raúl A. González-Pech1, Victor H. Beltran2, Bruno Lapeyre3,4,12, Pim Bongaerts5,6, Ira Cooke4, Manuel Aranda7, David G. Bourne2,8, Sylvain Forêt3,9, David J. Miller3,4, Madeleine J.H. van Oppen2,10, Christian R. Voolstra7, Mark A. Ragan1 & Cheong Xin Chan1,11 1234567890():,; Symbiosis between dinoflagellates of the genus Symbiodinium and reef-building corals forms the trophic foundation of the world’s coral reef ecosystems. Here we present the first draft genome of Symbiodinium goreaui (Clade C, type C1: 1.03 Gbp), one of the most ubiquitous endosymbionts associated with corals, and an improved draft genome of Symbiodinium kawagutii (Clade F, strain CS-156: 1.05 Gbp) to further elucidate genomic signatures of this symbiosis. Comparative analysis of four available Symbiodinium genomes against other dinoflagellate genomes led to the identification of 2460 nuclear gene families (containing 5% of Symbiodinium genes) that show evidence of positive selection, including genes involved in photosynthesis, transmembrane ion transport, synthesis and modification of amino acids and glycoproteins, and stress response. Further, we identify extensive sets of genes for meiosis and response to light stress. These draft genomes provide a foundational resource for advancing our understanding of Symbiodinium biology and the coral-algal symbiosis. 1 Institute for Molecular Bioscience, The University of Queensland, Brisbane, QLD 4072, Australia. 2 Australian Institute of Marine Science, Townsville, QLD 4810, Australia. 3 ARC Centre of Excellence for Coral Reef Studies, James Cook University, Townsville, QLD 4811, Australia. -
Delineating the Coral Triangle
Galaxea, Journal of Coral Reef Studies 11: 91-100(2009) Original paper Delineating the Coral Triangle J.E.N. VERON1, 2, *, Lyndon M. DEVANTIER1, 2, Emre TURAK1, 2, Alison L. GREEN3, Stuart KININMONTH2, Mary STAFFORD-SMITH1, and Nate PETERSON3 1 Coral Reef Research, 10 Benalla Road, Oak Valley, Townsville 4811, Australia 2 Australian Institute of Marine Science, MSO Townsville 4811, Australia 3 The Nature Conservancy, 51 Edmondstone Street, South Brisbane 4101, Australia * Corresponding author: J.E.N. Veron Email: [email protected] Communicated by Makoto Tsuchiya Abstract Spatial analyses of coral distributions at spe- Introduction cies level delineate the Coral Triangle and provide new insights into patterns of diversity and endemism around “If we look at a globe or a map of the Eastern Hemi the globe. This study shows that the Coral Triangle, an sphere, we shall perceive between Asia and Australia a area extending from the Philippines to the Solomon number of large and small islands, forming a connected Islands, has 605 zooxanthellate corals including 15 re- group distinct from those great masses of land and having gional endemics. This amounts to 76% of the world’s total little connexion with either of them. Situated upon the species complement, giving this province the world’s equator, and bathed in the tepid water of the great tropical highest conservation priority. Within the Coral Triangle, oceans, this region enjoys a climate more uniformly hot highest richness resides in the Bird’s Head Peninsula of and moist than almost any other part of the globe, and Indonesian Papua which hosts 574 species, with indi- teems with natural productions which are elsewhere vidual reefs supporting up to 280 species ha−1. -
Volume 19 Winter 2002 the Coral Hind, Lapu Lapu, Or Miniata
FREE ISSN 1045-3520 Volume 19 Winter 2002 Introducing a Zonal Based Natural Photo by Robert Fenner Filtration System for Reef Aquariums by Steve Tyree Quite a few natural based filtration systems have been devised by reef aquarists and scientists in the past twenty years. Some systems utilized algae to remove organic and inorganic pollutants from the reef aquarium; others utilized sediment beds. The natural filtration system that I have been researching and designing is drastically different from both of these types. No external algae are used. I believe that all the algae a functional reef requires are already growing in the reef, even if they are not apparent. They include micro-algae, turf algae, coralline algae, single-cell algae within photosynthetic corals, and cyanobacteria with photosynthetic capabilities. Most of the systems that I have set up to research this concept have not included sediment beds. All organic matter and pollutants are recycled and processed within the system by macro-organisms. Sediment beds have not been utilized to process excess Miniata Grouper, Cephalopholis miniata organic debris, but that does not prevent other aquarists from adding them. The main concept behind my system is the use of living sponges, sea squirts, and filter feeders for filtration. Sponges consume bacteria, can reach about twenty inches in length in the wild, and dissolved and colloidal organic material, micro-plankton, The Coral Hind, Lapu about half that in captivity. It is undoubtedly the most and fine particulate matter. Sea squirts consume large Lapu, or Miniata prized member of the genus for the aquarium trade.