Analysis of the Role of Polygalacturonase Inhibiting Proteins in the Interaction Between Plants and Nematodes
Total Page:16
File Type:pdf, Size:1020Kb
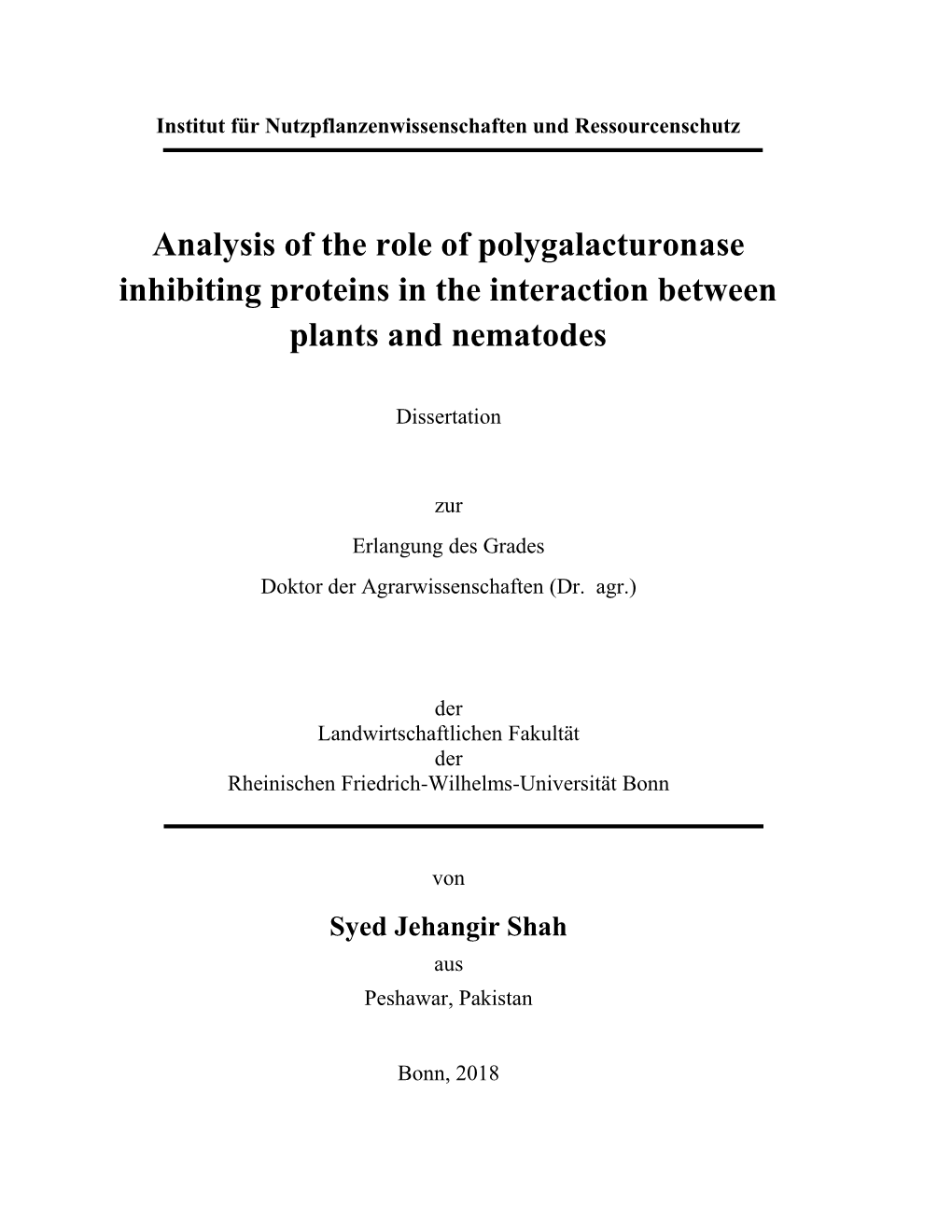
Load more
Recommended publications
-
JOURNAL of NEMATOLOGY Description of Heterodera
JOURNAL OF NEMATOLOGY Article | DOI: 10.21307/jofnem-2020-097 e2020-97 | Vol. 52 Description of Heterodera microulae sp. n. (Nematoda: Heteroderinae) from China a new cyst nematode in the Goettingiana group Wenhao Li1, Huixia Li1,*, Chunhui Ni1, Deliang Peng2, Yonggang Liu3, Ning Luo1 and Abstract 1 Xuefen Xu A new cyst-forming nematode, Heterodera microulae sp. n., was 1College of Plant Protection, Gansu isolated from the roots and rhizosphere soil of Microula sikkimensis Agricultural University/Biocontrol in China. Morphologically, the new species is characterized by Engineering Laboratory of Crop lemon-shaped body with an extruded neck and obtuse vulval cone. Diseases and Pests of Gansu The vulval cone of the new species appeared to be ambifenestrate Province, Lanzhou, 730070, without bullae and a weak underbridge. The second-stage juveniles Gansu Province, China. have a longer body length with four lateral lines, strong stylets with rounded and flat stylet knobs, tail with a comparatively longer hyaline 2 State Key Laboratory for Biology area, and a sharp terminus. The phylogenetic analyses based on of Plant Diseases and Insect ITS-rDNA, D2-D3 of 28S rDNA, and COI sequences revealed that the Pests, Institute of Plant Protection, new species formed a separate clade from other Heterodera species Chinese Academy of Agricultural in Goettingiana group, which further support the unique status of Sciences, Beijing, 100193, China. H. microulae sp. n. Therefore, it is described herein as a new species 3Institute of Plant Protection, Gansu of genus Heterodera; additionally, the present study provided the first Academy of Agricultural Sciences, record of Goettingiana group in Gansu Province, China. -
Proteomic Responses of Uninfected Tissues of Pea Plants Infected by Root-Knot Nematode, Fusarium and Downy Mildew Pathogens Al-S
PROTEOMIC RESPONSES OF UNINFECTED TISSUES OF PEA PLANTS INFECTED BY ROOT-KNOT NEMATODE, FUSARIUM AND DOWNY MILDEW PATHOGENS AL-SADEK MOHAMED SALEM GHAZALA A thesis submitted in partial fulfilment of the requirements of the University of the West of England, Bristol for the degree of Doctor of Philosophy. Department of Applied Sciences, University of the West of England, Bristol. December 2012 This copy has been supplied on the understanding that it is copyright material and that no quotation from the thesis may be published without proper acknowledgment. Al-Sadek Mohamed Salem Ghazala December 2012 Abstract Peas suffer from several diseases, and there is a need for accurate, rapid in-field diagnosis. This study used proteomics to investigate the response of pea plants to infection by the root knot nematode Meloidogyne hapla, the root rot fungus Fusarium solani and the downy mildew oomycete Peronospora viciae, and to identify potential biomarkers for diagnostic kits. A key step was to develop suitable protein extraction methods. For roots, the Amey method (Chuisseu Wandji et al., 2007), was chosen as the best method. The protein content of roots from plants with shoot infections by P. viciae was less than from non-infected plants. Specific proteins that had decreased in abundance were (1->3)-beta-glucanase, alcohol dehydrogenase 1, isoflavone reductase, malate dehydrogenase, mitochondrial ATP synthase subunit alpha, eukaryotic translation inhibition factor, and superoxide dismutase. No proteins increased in abundance in the roots of infected plants. For extraction of proteins from leaves, the Giavalisco method (Giavalisco et al., 2003) was best. The amount of protein in pea leaves decreased by age, and also following root infection by F. -
(Nematoda: Longidoridae) with Description of a New Species
Eur J Plant Pathol (2020) 158:59–81 https://doi.org/10.1007/s10658-020-02055-0 An integrative taxonomic study of the needle nematode complex Longidorus goodeyi Hooper, 1961 (Nematoda: Longidoridae) with description of a new species. Ruihang Cai & Tom Prior & Bex Lawson & Carolina Cantalapiedra-Navarrete & Juan E. Palomares-Rius & Pablo Castillo & Antonio Archidona-Yuste Received: 14 April 2020 /Revised: 8 June 2020 /Accepted: 19 June 2020 /Published online: 26 June 2020 # The Author(s) 2020 Abstract Needle nematodes are polyphagous root- and slightly offset by a depression with body contour, ectoparasites parasitizing a wide range of economically amphidial pouch with slightly asymmetrical lobes, important plants not only by directly feeding on root odontostyle 80.5–101.0 µm long, tail short and conoid cells, but also by transmitting nepoviruses. This study rounded. Longidorus panderaltum n. sp. is quite similar deciphers the diversity of the complex Longidorus to L. goodeyi and L. onubensis in major morphometrics goodeyi through integrative diagnosis method, based and morphology. However, differential morphology in on a combination of morphological, morphometrical, the tail shape of first-stage juvenile, phylogeny and multivariate analysis and molecular data. A new haplonet analyses indicate they are three distinct valid Longidorus species, Longidorus panderaltum n. sp. is species. This study defines those three species as mem- described and illustrated from a population associated bers of L. goodeyi complex group and reveals the taxo- with the rhizosphere of asphodel (Asphodelus ramosus nomical complexity of the genus Longidorus.This L.) in southern Spain. Morphologically, L. panderaltum L. goodeyi complex group demonstrated that the biodi- n. -
Morphological and Molecular Characterization of Heterodera Schachtii and the Newly Recorded Cyst Nematode, H
Plant Pathol. J. 34(4) : 297-307 (2018) https://doi.org/10.5423/PPJ.OA.12.2017.0262 The Plant Pathology Journal pISSN 1598-2254 eISSN 2093-9280 ©The Korean Society of Plant Pathology Research Article Open Access Morphological and Molecular Characterization of Heterodera schachtii and the Newly Recorded Cyst Nematode, H. trifolii Associated with Chinese Cabbage in Korea Abraham Okki Mwamula1†, Hyoung-Rai Ko2†, Youngjoon Kim1, Young Ho Kim1, Jae-Kook Lee2, and Dong Woon Lee 1* 1Department of Ecological Science, Kyungpook National University, Sangju 37224, Korea 2Crop Protection Division, National Institute of Agricultural Sciences, Rural Development Administration, Wanju 55365, Korea (Received on December 23, 2017; Revised on March 6, 2018; Accepted on March 13, 2018) The sugar beet cyst nematode, Heterodera schachtii population whereas those of H. schachtii were strongly is a well known pathogen on Chinese cabbage in the detected in H. schachtii monoxenic cultures. Thus, this highland fields of Korea. However, a race of cyst form- study confirms the coexistence of the two species in ing nematode with close morphological resemblance to some Chinese cabbage fields; and the presence of H. tri- H. trifolii was recently isolated from the same Chinese folii in Korea is reported here for the first time. cabbage fields. Morphological species differentiation between the two cyst nematodes is challenging, with Keywords : infective juvenile, morphometrics, vulval cone only minor differences between them. Thus, this study described the newly intercepted H. trifolii population, Handling Associate Editor : Lee, Yong Hoon and reviewed morphological and molecular charac- teristics conceivably essential in differentiating the two nematode species. -
Morphological and Molecular Characterisation of Paralongidorus Rex Andrássy, 1986 (Nematoda: Longidoridae) from Poland and Ukraine
Eur J Plant Pathol (2015) 141:385–395 DOI 10.1007/s10658-014-0550-2 Morphological and molecular characterisation of Paralongidorus rex Andrássy, 1986 (Nematoda: Longidoridae) from Poland and Ukraine Franciszek Wojciech Kornobis & Solomija Susulovska & Andrij Susulovsky & Sergei A. Subbotin Accepted: 7 October 2014 /Published online: 17 October 2014 # The Author(s) 2014. This article is published with open access at Springerlink.com Abstract Paralongidorus rex was found for the first profiles with five enzymes are given. Additionally, in- time in Poland and Ukraine. This paper describes fe- formation on new host plants and map of distribution for males and juveniles from four populations of this spe- P. re x are provided. The new record of this nematode cies on the basis of morphology and morphometrics and species, previously identified as Paralongidorus sp. provides molecular characterization using 18S, ITS1 (GenBank: AY601582) from Slovakia, is defined based and D2-D3 expansion segments of 28S rRNA gene on comparison of sequences of the D2-D3 expansion sequences. Morphometrically, females from these segments of 28S rRNA gene. Finally, remarks on the populations differed slighty in V ratio (means in four potential importance of this species in grapevine pro- populations: 41.9; 42.7; 46.1; 46.8) and odontostylet duction are given. length (166.6; 170.6; 191.5; 193.2). Phylogenetic anal- ysis showed that P. re x had a sister relationship with Keywords Paralongidorus rex . Morphometrics . P. iranicus. PCR-D2-D3 of 28S-RFLP diagnostic D2-D3 of 28S rRNA gene . ITS1 rRNA gene . 18S rRNA gene . RFLP F. W. Kornobis (*) Department of Zoology, Institute of Plant Protection- National Research Institute, Władysława Węgorka 20, 60-318 Poznań, Nematodes of the family Longidoridae are obligatory Poland plant parasites and are considered as economically e-mail: [email protected] important pests. -
Description and Molecular Characterisation of Paralongidorus Litoralis Sp.N.Andp
Nematology, 2008, Vol. 10(1), 87-101 Description and molecular characterisation of Paralongidorus litoralis sp.n.andP. paramaximus Heyns, 1965 (Nematoda: Longidoridae) from Spain Juan E. PALOMARES-RIUS 1,SergeiA.SUBBOTIN 2,3,BlancaB.LANDA 1, ∗ Nicola VOVLAS 4 and Pablo CASTILLO 1, 1 Institute of Sustainable Agriculture, Spanish National Research Council (CSIC), Alameda del Obispo s/n, Apdo. 4084, 14080 Córdoba, Spain 2 Plant Pest Diagnostics Center, California Department of Food and Agriculture, 3294 Meadowview Road, Sacramento, CA 95832-1448, USA 3 Center of Parasitology of A.N. Severtsov Institute of Ecology and Evolution of the Russian Academy of Sciences, Leninskii Prospect 33, Moscow, 117071, Russia 4 Istituto per la Protezione delle Piante, Sezione di Bari, Consiglio Nazionale delle Ricerche, (CNR), Via G. Amendola 165/A, 70126 Bari, Italy Received: 17 July 2007; revised: 23 August 2007 Accepted for publication: 23 August 2007 Summary – Paralongidorus litoralis sp. n., a new bisexual species of the genus, is described and illustrated by light microscopy, scanning electron microscopy and molecular studies from specimens collected in a coastal sand dune soil around roots of lentisc (Pistacia lentiscus L.) from Zahara de los Atunes (Cadiz), southern Spain. Paralongidorus litoralis sp. n. is characterised by the large body size (7.5-10.0 mm), a rounded lip region, clearly offset from the body by a collar-like constriction, and bearing a very large stirrup-shaped, amphidial fovea, with conspicuous slit-like aperture, a very long and flexible odontostyle ca 190 µm long, guiding ring located at 35 µm from anterior end, and males with spicules ca 70 µm long. -
JOURNAL of NEMATOLOGY Morphological And
JOURNAL OF NEMATOLOGY Article | DOI: 10.21307/jofnem-2020-098 e2020-98 | Vol. 52 Morphological and molecular characterization of Heterodera dunensis n. sp. (Nematoda: Heteroderidae) from Gran Canaria, Canary Islands Phougeishangbam Rolish Singh1,2,*, Gerrit Karssen1, 2, Marjolein Couvreur1 and Wim Bert1 Abstract 1Nematology Research Unit, Heterodera dunensis n. sp. from the coastal dunes of Gran Canaria, Department of Biology, Ghent Canary Islands, is described. This new species belongs to the University, K.L. Ledeganckstraat Schachtii group of Heterodera with ambifenestrate fenestration, 35, 9000, Ghent, Belgium. presence of prominent bullae, and a strong underbridge of cysts. It is characterized by vermiform second-stage juveniles having a slightly 2National Plant Protection offset, dome-shaped labial region with three annuli, four lateral lines, Organization, Wageningen a relatively long stylet (27-31 µm), short tail (35-45 µm), and 46 to 51% Nematode Collection, P.O. Box of tail as hyaline portion. Males were not found in the type population. 9102, 6700, HC, Wageningen, Phylogenetic trees inferred from D2-D3 of 28S, partial ITS, and 18S The Netherlands. of ribosomal DNA and COI of mitochondrial DNA sequences indicate *E-mail: PhougeishangbamRolish. a position in the ‘Schachtii clade’. [email protected] This paper was edited by Keywords Zafar Ahmad Handoo. 18S, 28S, Canary Islands, COI, Cyst nematode, ITS, Gran Canaria, Heterodera dunensis, Plant-parasitic nematodes, Schachtii, Received for publication Systematics, Taxonomy. September -
DNA Barcoding Evidence for the North American Presence of Alfalfa Cyst Nematode, Heterodera Medicaginis Tom Powers
University of Nebraska - Lincoln DigitalCommons@University of Nebraska - Lincoln Papers in Plant Pathology Plant Pathology Department 8-4-2018 DNA barcoding evidence for the North American presence of alfalfa cyst nematode, Heterodera medicaginis Tom Powers Andrea Skantar Timothy Harris Rebecca Higgins Peter Mullin See next page for additional authors Follow this and additional works at: https://digitalcommons.unl.edu/plantpathpapers Part of the Other Plant Sciences Commons, Plant Biology Commons, and the Plant Pathology Commons This Article is brought to you for free and open access by the Plant Pathology Department at DigitalCommons@University of Nebraska - Lincoln. It has been accepted for inclusion in Papers in Plant Pathology by an authorized administrator of DigitalCommons@University of Nebraska - Lincoln. Authors Tom Powers, Andrea Skantar, Timothy Harris, Rebecca Higgins, Peter Mullin, Saad Hafez, Zafar Handoo, Tim Todd, and Kirsten S. Powers JOURNAL OF NEMATOLOGY Article | DOI: 10.21307/jofnem-2019-016 e2019-16 | Vol. 51 DNA barcoding evidence for the North American presence of alfalfa cyst nematode, Heterodera medicaginis Thomas Powers1,*, Andrea Skantar2, Tim Harris1, Rebecca Higgins1, Peter Mullin1, Saad Hafez3, Abstract 2 4 Zafar Handoo , Tim Todd & Specimens of Heterodera have been collected from alfalfa fields 1 Kirsten Powers in Kearny County, Kansas and Carbon County, Montana. DNA 1University of Nebraska-Lincoln, barcoding with the COI mitochondrial gene indicate that the species is Lincoln NE 68583-0722. not Heterodera glycines, soybean cyst nematode, H. schachtii, sugar beet cyst nematode, or H. trifolii, clover cyst nematode. Maximum 2 Mycology and Nematology Genetic likelihood phylogenetic trees show that the alfalfa specimens form a Diversity and Biology Laboratory sister clade most closely related to H. -
Histopathology of Brassica Oleracea Var. Capitata Subvar
Türk. entomol. derg., 2012, 36 (3): 301-309 ISSN 1010-6960 Orijinal araştırma (Original article) Histopathology of Brassica oleracea var. capitata subvar. alba infected with Heterodera cruciferae Franklin, 1945 (Tylenchida: Heteroderidae) Heterodera cruciferae Franklin, 1945 (Tylenchida: Heteroderidae) ile bulaşık Brassica oleracea var. capitata subvar. alba`nın histopatojisi Sevilhan MENNAN1* Zafar A. HANDOO2 Summary Anatomical changes induced by the cabbage cyst nematode (Heterodera cruciferae) have been insufficiently characterized. Here these changes were described in the root tissues of white head cabbage variety (Yalova F1) commonly grown in the Black Sea region of Turkey, where cabbage-growing areas are heavily infested. In glasshouse experiments conducted at 20 degrees C, susceptible white head cabbage seedlings were inoculated with 0 (untreated control) or 1000 juveniles/300 ml soil. Three, 6, 12, 24, 48, 72 h and 30 days after inoculations, two plants from each treatment were removed, embedded in paraffin by using microwave technique and then examined by photomicrography. Second-stage passed through the vascular system after root penetration and they started to feed as sedentary. In cross section of the roots, large cells in the cortex of infected plants were filled with moderately dense cytoplasm and the walls were heavily stained and ruptured. In longitudinal section, internal walls were perforated. Syncytia that had different degrees of vacuolization, and syncytial nuclei were hypertrophied and deeply indented. Contained conspicuous nucleoli were noticeable 24 h after inoculation. Syncytia originating from endodermal cells possessed ruptured walls around the feeding site of the developing juvenile. White females were observed on the roots 30 days after inoculation, a time at which plant height was reduced and root proliferation increased. -
Functional Diversity of Soil Nematodes in Relation to the Impact of Agriculture—A Review
diversity Review Functional Diversity of Soil Nematodes in Relation to the Impact of Agriculture—A Review Stela Lazarova 1,* , Danny Coyne 2 , Mayra G. Rodríguez 3 , Belkis Peteira 3 and Aurelio Ciancio 4,* 1 Institute of Biodiversity and Ecosystem Research, Bulgarian Academy of Sciences, 2 Y. Gagarin Str., 1113 Sofia, Bulgaria 2 International Institute of Tropical Agriculture (IITA), Kasarani, Nairobi 30772-00100, Kenya; [email protected] 3 National Center for Plant and Animal Health (CENSA), P.O. Box 10, Mayabeque Province, San José de las Lajas 32700, Cuba; [email protected] (M.G.R.); [email protected] (B.P.) 4 Consiglio Nazionale delle Ricerche, Istituto per la Protezione Sostenibile delle Piante, 70126 Bari, Italy * Correspondence: [email protected] (S.L.); [email protected] (A.C.); Tel.: +359-8865-32-609 (S.L.); +39-080-5929-221 (A.C.) Abstract: The analysis of the functional diversity of soil nematodes requires detailed knowledge on theoretical aspects of the biodiversity–ecosystem functioning relationship in natural and managed terrestrial ecosystems. Basic approaches applied are reviewed, focusing on the impact and value of soil nematode diversity in crop production and on the most consistent external drivers affecting their stability. The role of nematode trophic guilds in two intensively cultivated crops are examined in more detail, as representative of agriculture from tropical/subtropical (banana) and temperate (apple) climates. The multiple facets of nematode network analysis, for management of multitrophic interactions and restoration purposes, represent complex tasks that require the integration of different interdisciplinary expertise. Understanding the evolutionary basis of nematode diversity at the field Citation: Lazarova, S.; Coyne, D.; level, and its response to current changes, will help to explain the observed community shifts. -
Molecular Characterization of Cyst Nematode Species (Heterodera Spp.) from the Mediterranean Basin Using Rflps and Sequences of ITS-Rdna
J. Phytopathology 152, 229–234 (2004) Ó 2004 Blackwell Verlag, Berlin ISSN 0931-1785 Crop Protection Department, Agricultural Research Centre, Merelbeke, Belgium Molecular Characterization of Cyst Nematode Species (Heterodera spp.) from the Mediterranean Basin using RFLPs and Sequences of ITS-rDNA M.Madani 1, N.Vovlas 2, P.Castillo 3, S. A.Subbotin 4 and M.Moens 1,51,5 AuthorsÕ addresses: 1Crop Protection Department, Agricultural Research Centre, Burg, Van Gansberghelaan 96, 9820 Merelbeke, Belgium; 2Istituto per la Protezione delle Piante, Sezione di Bari: Nematologia Agraria, Consiglio Nazionale delle Ricerche (C.N.R.), Via G. Amendola 165/A, 70126 Bari, Italy; 3Instituto de Agricultura Sostenible, Consejo Superior de Investigaciones Cientificas (C.S.I.C.), Apdo. 4084, 14080 Cordoba, Spain; 4Institute of Parasitology of the Russian Academy of Sciences, Leninskii Prospect 33, Moscow 119071, Russia; 5University of Gent, Laboratory for Agrozoology, Coupure 555, 9000 Gent, Belgium (correspondence to S. A. Subbotin. E-mail: [email protected]) With 2 figures Received August 6, 2003; accepted February 11, 2004 Keywords: Heterodera carotae, Heterodera ciceri, Heterodera fici, Heterodera filipjevi, Heterodera goettingiana, Heterodera hordecalis, Heterodera humuli, Heterodera mediterranea, Heterodera ripae, Heterodera schachtii, internal transcribed spacer region, phylogenetic relationships, molecular identification Abstract plant growth and yield may be suppressed (Baldwin Fifteen populations of cyst-forming nematodes belong- and Mundo Ocampo, 1991). ing to 11 known and one unidentified species collected Currently, the genus Heterodera contains more than in countries bordering the Mediterranean Sea were 60 species (Wouts and Baldwin, 1998). In the Mediterra- studied using polymerase chain reaction restriction nean Basin several cyst nematode species have been fragment length polymorphism (PCR–RFLP) and reported on herbaceous and woody plants (Greco and internal transcribed spacer (ITS)-rDNA sequences. -
WO 2008/079012 Al
(12) INTERNATIONAL APPLICATION PUBLISHED UNDER THE PATENT COOPERATION TREATY (PCT) (19) World Intellectual Property Organization International Bureau (43) International Publication Date PCT (10) International Publication Number 3 July 2008 (03.07.2008) WO 2008/079012 Al (51) International Patent Classification: GB Nijmegen (NL). LANDEWEERT, Renske [NL/NL]; C12Q 1/68 (2006.01) Kerkeland 40, NL-6862 VB Oosterbeek (NL). HERMAN, Henri [NL/NL]; Kerkeland 40, NL-6862 VB Oosterbeek (21) International Application Number: (NL). BARKER, Jaap [NL/NL]; Geertjesweg 122, PCT/NL2007/050700 NL-6704 PD Wageningen (NL). (22) International Filing Date: (74) Agent: HATZMANN, M.J.; Vereenigde, Johan de Witt- 2 1 December 2007 (21.12.2007) laan 7, NL-2517 JR Den Haag (NL). (81) Designated States (unless otherwise indicated, for every (25) Filing Language: English kind of national protection available): AE, AG, AL, AM, AT,AU, AZ, BA, BB, BG, BH, BR, BW, BY,BZ, CA, CH, (26) Publication Language: English CN, CO, CR, CU, CZ, DE, DK, DM, DO, DZ, EC, EE, EG, ES, FI, GB, GD, GE, GH, GM, GT, HN, HR, HU, ID, IL, (30) Priority Data: IN, IS, JP, KE, KG, KM, KN, KP, KR, KZ, LA, LC, LK, 06077326.4 27 December 2006 (27.12.2006) EP LR, LS, LT, LU, LY,MA, MD, ME, MG, MK, MN, MW, MX, MY, MZ, NA, NG, NI, NO, NZ, OM, PG, PH, PL, (71) Applicants (for all designated States except US): WA- PT, RO, RS, RU, SC, SD, SE, SG, SK, SL, SM, SV, SY, GENINGEN UNIVERSITEIT [NL/NL]; Costerweg TJ, TM, TN, TR, TT, TZ, UA, UG, US, UZ, VC, VN, ZA, 50, NL-6701 BH Wageningen (NL).