Realization of X-Ray Telescopes—From Design to Performance
Total Page:16
File Type:pdf, Size:1020Kb
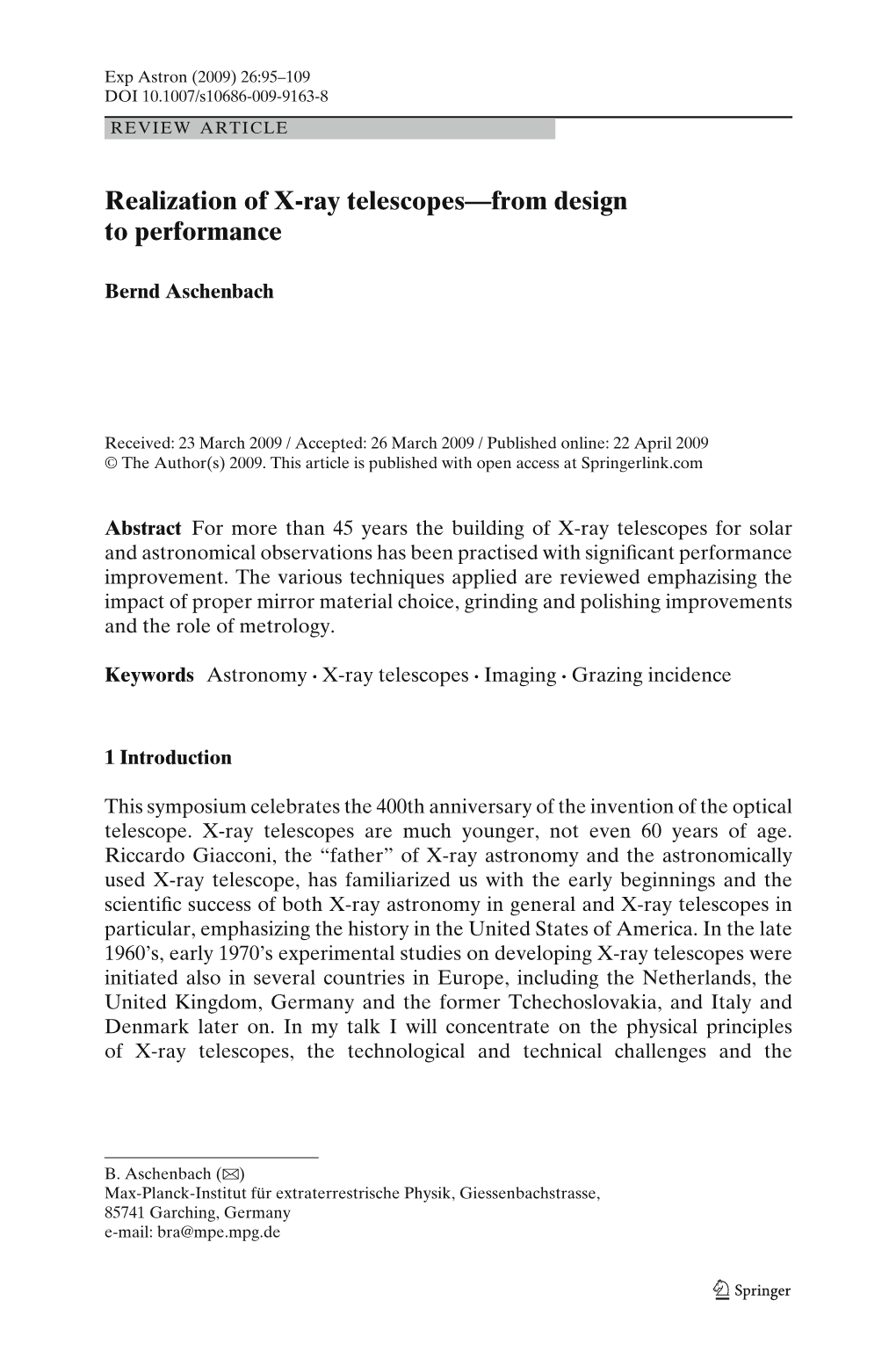
Load more
Recommended publications
-
GRIPS-Gamma-Ray Imaging, Polarimetry and Spectroscopy
Experimental Astronomy manuscript No. (will be inserted by the editor) GRIPS - Gamma-Ray Imaging, Polarimetry and Spectroscopy www.grips-mission.eu? Jochen Greiner · Karl Mannheim · Felix Aharonian · Marco Ajello · Lajos G. Balasz · Guido Barbiellini · Ronaldo Bellazzini · Shawn Bishop · Gennady S. Bisnovatij-Kogan · Steven Boggs · Andrej Bykov · Guido DiCocco · Roland Diehl · Dominik Els¨asser · Suzanne Foley · Claes Fransson · Neil Gehrels · Lorraine Hanlon · Dieter Hartmann · Wim Hermsen · Wolfgang Hillebrandt · Rene Hudec · Anatoli Iyudin · Jordi Jose · Matthias Kadler · Gottfried Kanbach · Wlodek Klamra · J¨urgenKiener · Sylvio Klose · Ingo Kreykenbohm · Lucien M. Kuiper · Nikos Kylafis · Claudio Labanti · Karlheinz Langanke · Norbert Langer · Stefan Larsson · Bruno Leibundgut · Uwe Laux · Francesco Longo · Kei'ichi Maeda · Radoslaw Marcinkowski · Martino Marisaldi · Brian McBreen · Sheila McBreen · Attila Meszaros · Ken'ichi Nomoto · Mark Pearce · Asaf Peer · Elena Pian · Nikolas Prantzos · Georg Raffelt · Olaf Reimer · Wolfgang Rhode · Felix Ryde · Christian Schmidt · Joe Silk · Boris M. Shustov · Andrew Strong · Nial Tanvir · Friedrich-Karl Thielemann · Omar Tibolla · David Tierney · Joachim Tr¨umper · Dmitry A. Varshalovich · J¨orn Wilms · Grzegorz Wrochna · Andrzej Zdziarski · Andreas Zoglauer Received: 21 April 2011 / Accepted: 2011 ? See this Web-site for the author's affiliations. Jochen Greiner Karl Mannheim MPI f¨urextraterrestrische Physik Inst. f. Theor. Physik & Astrophysik, Univ. W¨urzburg arXiv:1105.1265v1 [astro-ph.HE] 6 May 2011 85740 Garching, Germany 97074 W¨urzburg,Germany Tel.: +49-89-30000-3847 Tel.: +49-931-318-500 E-mail: [email protected] E-mail: [email protected] 2 Abstract We propose to perform a continuously scanning all-sky survey from 200 keV to 80 MeV achieving a sensitivity which is better by a factor of 40 or more compared to the previous missions in this energy range (COMPTEL, INTEGRAL; see Fig. -
The Cosmic X-Ray Background
The Cosmic X-Ray Background Steven M. Kahn Kavli Institute for Particle Astrophysics and Cosmology Stanford University 1 Outline of Lectures Lecture I: * Historical Introduction and General Characteristics of the CXB. * Contributions from Discrete Source Classes * Spectral Paradoxes Lecture II: * The CXB and Large Scale Structure * The Galactic Contributions to the CXB * The CXB and the Cosmic Web 2 I. Historical Introduction and General Characteristics of the CXB. 3 Historical Introduction * The “birth” of the field of X-ray astronomy is usually associated with the flight of a particular rocket experiment in 1962 that yielded the detection of the first non-solar cosmic X-ray source: Scorpius X-1. (Giacconi et al. 1962) * That same rocket experiment also yielded the discovery of an apparent diffuse component of X-radiation, the Cosmic X-ray Background (CXB). (N.B. This was well in advance of the discovery of the Cosmic Microwave Background by Pensias and Wilson!) * In the ensuing 40 some odd years, our understanding of the X-ray Universe has progressed considerably. X-rays have now been detected from virtually all classes of astronomical systems, ranging from normal stars to the most distant galaxies. * Nevertheless, the precise origin of the CXB remains puzzling. This has been one of the great mysteries of high energy astrophysics! 4 Historical Introduction 5 Historical Introduction * “The diffuse character of the observed background radiation does not permit a positive determination of its nature and origin. However, the apparent absorption coefficient in mica and the altitude dependence is consistent with radiation of about the same wavelength responsible for the peak. -
Chandra Was Launched Aboard Space Shuttle Columbia on July 23, 1999!!! Crew Lost During Re-Entry Modern X-Ray Telescopes and Detectors
Chandra was launched aboard Space Shuttle Columbia on July 23, 1999!!! Crew Lost During Re-Entry Modern X-ray Telescopes and Detectors •X-ray Telescopes •X-ray Instruments •Some early highlights •Observations •Data characteristics •Calibration •Analysis X-ray Telescope: The advantages • Achieve 2-D imaging – Separate sources – Study morphology of extended sources – Simultaneously measure both source and local background • Reduce the background Æ increase the source 1/2 detection sensitivity: S/N ~ Fs t/(Fst+ ASbt) –t –exposure time –Fs – source count flux –A –source detection area –Sb – background surface brightness: Detector + sky background • Facilitate high-resolution dispersive spectrometers X-ray Telescope: Focusing mechanism External reflection at small grazing angles - an analogy of skipping stones on water •Snell’s law: sinφr=sinφi/n, where the index of refraction n=1-δ+iβ • External reflection occurs with sinφr > 1 Æ 1/2 The critical grazing angle θ = π/2- φi ~ (2δ) 1/2 (δ ∝ ne /E << 1) Focusing mechanism (cont.) • The critical angle (effective collecting area) decreases with increasing photon energy • High Z materials allow for reflecting high energy photon with the same grazing angle X-ray Telescope: Hans Wolter Configuraions • A Paraboloid gives a perfect image for on-axis rays. But it gives a coma blur of equivalent image size proportional to the off-axis angle. • Wolter showed that two reflections were needed to eliminate the coma. • A Paraboloid-Hyperboloid combination proves to be the most useful in X-ray astronomy. X-ray Telescopes • First used to observe the Solar corona • Then transferred to general astronomy with HEAO-2 (Einstein Observatory), launched in 1978: – imaged X-rays in 0.5-4.0 keV. -
Highlights and Discoveries from the Chandra X-Ray Observatory1
Highlights and Discoveries from the Chandra X-ray Observatory1 H Tananbaum1, M C Weisskopf2, W Tucker1, B Wilkes1 and P Edmonds1 1Smithsonian Astrophysical Observatory, 60 Garden Street, Cambridge, MA 02138. 2 NASA/Marshall Space Flight Center, ZP12, 320 Sparkman Drive, Huntsville, AL 35805. Abstract. Within 40 years of the detection of the first extrasolar X-ray source in 1962, NASA’s Chandra X-ray Observatory has achieved an increase in sensitivity of 10 orders of magnitude, comparable to the gain in going from naked-eye observations to the most powerful optical telescopes over the past 400 years. Chandra is unique in its capabilities for producing sub-arcsecond X-ray images with 100-200 eV energy resolution for energies in the range 0.08<E<10 keV, locating X-ray sources to high precision, detecting extremely faint sources, and obtaining high resolution spectra of selected cosmic phenomena. The extended Chandra mission provides a long observing baseline with stable and well-calibrated instruments, enabling temporal studies over time-scales from milliseconds to years. In this report we present a selection of highlights that illustrate how observations using Chandra, sometimes alone, but often in conjunction with other telescopes, have deepened, and in some instances revolutionized, our understanding of topics as diverse as protoplanetary nebulae; massive stars; supernova explosions; pulsar wind nebulae; the superfluid interior of neutron stars; accretion flows around black holes; the growth of supermassive black holes and their role in the regulation of star formation and growth of galaxies; impacts of collisions, mergers, and feedback on growth and evolution of groups and clusters of galaxies; and properties of dark matter and dark energy. -
16Th HEAD Meeting Session Table of Contents
16th HEAD Meeting Sun Valley, Idaho – August, 2017 Meeting Abstracts Session Table of Contents 99 – Public Talk - Revealing the Hidden, High Energy Sun, 204 – Mid-Career Prize Talk - X-ray Winds from Black Rachel Osten Holes, Jon Miller 100 – Solar/Stellar Compact I 205 – ISM & Galaxies 101 – AGN in Dwarf Galaxies 206 – First Results from NICER: X-ray Astrophysics from 102 – High-Energy and Multiwavelength Polarimetry: the International Space Station Current Status and New Frontiers 300 – Black Holes Across the Mass Spectrum 103 – Missions & Instruments Poster Session 301 – The Future of Spectral-Timing of Compact Objects 104 – First Results from NICER: X-ray Astrophysics from 302 – Synergies with the Millihertz Gravitational Wave the International Space Station Poster Session Universe 105 – Galaxy Clusters and Cosmology Poster Session 303 – Dissertation Prize Talk - Stellar Death by Black 106 – AGN Poster Session Hole: How Tidal Disruption Events Unveil the High 107 – ISM & Galaxies Poster Session Energy Universe, Eric Coughlin 108 – Stellar Compact Poster Session 304 – Missions & Instruments 109 – Black Holes, Neutron Stars and ULX Sources Poster 305 – SNR/GRB/Gravitational Waves Session 306 – Cosmic Ray Feedback: From Supernova Remnants 110 – Supernovae and Particle Acceleration Poster Session to Galaxy Clusters 111 – Electromagnetic & Gravitational Transients Poster 307 – Diagnosing Astrophysics of Collisional Plasmas - A Session Joint HEAD/LAD Session 112 – Physics of Hot Plasmas Poster Session 400 – Solar/Stellar Compact II 113 -
Eyes for Gamma Rays” Though the Major Peaks Suggest a Periodic- Whether These Are Truly Gamma-Ray Bursts for a Description of This System)
sion of regularity and slow evolution in the They suggested that examination of the Vela exe-atmospheric nuclear detonation. Surpris- universe persisted into the 1960s. data might disclose evidence of bursts of ingly, however, the survey soon revealed that The feeling that transient cosmic events gamma rays at times close to the appearance the gamma-ray instruments on widely sepa- were rare was certainly prevalent in 1959 of supernovae. Such searches were con- rated satellites had sometimes responded when summit meetings were being held be- ducted; however, no distinctive signals were almost identically. Some of these events were tween England, the United States, and found. attributable to solar flare activity. However, Russia to discuss a nuclear test-ban treaty. On the other hand, there was evidence of one particularly distinctive event was dis- One key issue was the ability to detect treaty variability that had been ignored. For exam- covered for which a solar origin seemed violations unambiguously. A leading ple, the earliest x-ray data from small rocket inconsistent. Fortunately, the characteristics proposal for the detection of exo-at- probes and from satellites were often found of this event did not at all resemble those of a mospheric nuclear explosions was the use of to disagree significantly. The quality of the nuclear detonation, and thus the event did satellites with instruments that included de- data, rather than actual variations in the not create concern of a possible test-ban tectors sensitive to the gamma rays emitted sources, was suspected as the reason for treaty violation. by the explosion as well as those emitted these discrepancies. -
A Stacked Prism Lens Concept for Next-Generation Hard X-Ray Telescopes
A Stacked Prism Lens Concept for Next-Generation Hard X-Ray Telescopes WUJUN MI Doctoral Thesis Stockholm, Sweden 2019 KTH Fysik TRITA-SCI-FOU 2019:41 SE-106 91 Stockholm ISBN 978-91-7873-286-9 SWEDEN Akademisk avhandling som med tillstånd av Kungl Tekniska högskolan framlägges till offentlig granskning för avläggande av teknologie doktorsexamen i fysik fredagen den 27 september 2019 klockan 10.00 i E3, Osquars backe 14, Kungliga Tekniska Högskolan, Stockholm. © Wujun Mi, September 2019 Tryck: Universitetsservice US AB iii Abstract Over the past half century, the focusing X-ray telescope has played a very prominent role in X-ray astronomy at the frontier of fundamental physics. The finer angular resolution and increased effective area have enabled more and more exciting discoveries and detailed studies of the high-energy universe, including the cosmic X-ray background (CXB) radiation, black holes in active galactic nuclei (AGN), galaxy clusters, supernova remnants, and so on. At present, nearly all the state-of-the-art focusing X-ray telescopes are based on Wolter-I optics or its variations, for which the throughput is severely restricted by the mirror’s surface roughness, figure error, alignment error, and so on. Within the course of this work, we have developed a novel point-focusing refractive lens, the stacked prism lens (SPL), which is built by stacking disks embedded with various number of prismatic rings. As a Fresnel-like X-ray lens, it could provide a significantly higher efficiency and larger effective aper- ture than the conventional compound refractive lenses (CRLs). The aim of this thesis is to demonstrate the feasibility of the stacked prism lens and investigate the application to a next-generation hard X-ray telescope. -
Ii. Future Missions (A) X-Ray and Gamma-Ray Missions
II. FUTURE MISSIONS (A) X-RAY AND GAMMA-RAY MISSIONS Downloaded from https://www.cambridge.org/core. IP address: 170.106.35.76, on 29 Sep 2021 at 08:47:06, subject to the Cambridge Core terms of use, available at https://www.cambridge.org/core/terms. https://doi.org/10.1017/S0252921100076909 RONTGEN SATELLITE J. TRUMPER MPI fur Extraterrestrische Physik, D-8O46 Garching-bei-Miinchen The ROSAT launch on June 1, 1990 happened to be after the IAU Colloquium No. 123 before the deadline for submitting manuscripts. I therefore take the liberty to deviate grossly from the manuscript of my talk and give a short up to date mission summary. A more complete description of the mission can be found in References 1 and 2. The launch of the Delta II rocket was perfect and the orbital parameters reached are very close to nominal: height 580 km, inclination 53°. The predicted satellite's lifetime in this orbital is at least 10 years. The switch-on of the spacecraft and instrument subsystems could be completed without any losses. All systems are in good health. ROSAT carries two instruments: — a large Wolter telescope covering the energy range 0.1-2.4 keV with two position sensitive proportional counters (PSPC) and one high resolution imager (HRI) and — Wolter-Schwarzschild-telescope with two channel plate detectors covering the adjacent XUV-range. The novel features of the X-ray telescope are: — large collecting power — good spectral resolution of the PSPC's: AE/E ~ 0.4 at 1 keV; four colours — low background of the PSPC's: 3 x 10-5 cts/arcmin2 sec — high angular resolution with the HRI: < 4 arcsec half power width (PSPC ~ 25 arcsec) The ROSAT mission comprises three phases: After the initial calibration and verification phase an all sky survey is carried out during half a year, followed by a period of pointed observations. -
Metrology of X-Ray Optics in Pursuit of Perfection
Metrology of X-ray Optics In Pursuit of Perfection Peter Z. Takacs Brookhaven National Laboratory Laboratory for Forensic Metrology Center for Archaeo-metrology MEADOW 2013 Workshop, ICTP Trieste, Italy 28-30 October 2013 1 Roadmap § One man's journey in pursuit of the perfect surface • A retrospective on how profilometry has improved the state-of-the-art of fabrication of SR optics over the past 30+ years. § Brief history of x-ray optics § Surface finish metrology § Surface figure metrology This presentation has been authored by Brookhaven Science Associates, LLC under Contract No. DE-AC02-98CH10886 with the U.S. Department of Energy. DISCLAIMER Reference herein to any specific commercial product, process, or service by trade name, trademark, manufacturer, or otherwise, does not necessarily constitute or imply its endorsement, recommendation, or favoring by Brookhaven National Laboratory, the Department of Energy, or the United States Government. The views and opinions expressed herein do not necessarily state or reflect those of Brookhaven National Laboratory, the Department of Energy, or the United States Government, and may not be used for advertising or product endorsement purposes. 2 Giovanni Sostero – friend and colleague 3 Reflective x-ray optics BRIEF HISTORICAL INTRODUCTION 4 Brief history of significant events in x-ray science Autoradiography imaging Crystal structure Surface reflection 1910 1920 1930 1895 1900 1912 1918 1923 1899 Recognizes Total external reflection X-rays are electromagnetic measurements as demonstrated waves (Haga&Wind) total external reflection Röntgen discovers x-rays Diffraction from crystals A. Einstein W. H. Bragg W. L. Bragg (1879-1955) Arthur Holly Compton Max von Laue (1862-1942) (1890-1971) (1892-1962) (1879-1960) Wilhelm Conrad Röntgen (1845-1923) 5 A brief history – cont. -
High Energy Properties of X-Ray Sources Observed with Bepposax
1 High energy properties of X–ray sources observed with BeppoSAX F. Fronteraa,b, D. Dal Fiumeb, G. Malagutib, L. Nicastrob, M. Orlandinib, E. Palazzib, E. Pianb, F. Favatac, A. Santangelod, a Dipartimento di Fisica, Universit`adi Ferrara, Via Paradiso 12, 44100 Ferrara, Italy b Istituto Tecnologie e Studio Radiazioni Extraterrestri (TeSRE), C.N.R., via Gobetti 101, 40129 Bologna, Italy c Astrophysics Division, Space Science Department of ESA, ESTEC Keplerlaan 1, 2200 AG Noordwijk, The Netherlands d Istituto Fisica Cosmica e Applicazioni all’Informatica (IFCAI), C.N.R., via La Malfa 153, 90146 Palermo, Italy We report on highlight results on celestial sources observed in the high energy band (> 20 keV) with BeppoSAX . In particular we review the spectral properties of sources that belong to different classes of objects, i.e., stellar coronae (Algol), supernova remnants (Cas A), low mass X–ray binaries (Cygnus X–2 and the X–ray burster GS1826–238), black hole candidates (Cygnus X–1) and Active Galactic Nuclei (Mkn 3). We detect, for the first 44 time, the broad-band spectrum of a stellar corona up to 100 keV; for Cas A we report upper limits to the Ti line intensities that are lower than those available to date; for Cyg X–2 we report the evidence of a high energy component; we report a clear detection of a broad Fe K line feature from Cyg X–1 in soft state and during its transition to hard state; Mkn 3 is one of several Seyfert 2 galaxies detected with BeppoSAX at high energies, for which Compton scattering process is important. -
RICCARDO GIACCONI Associated Universities, Inc., Suite 730, 1400 16Th St., NW, Washington, DC 20036, USA, and Johns Hopkins University, 3400 N
THE DAWN OF X-RAY ASTRONOMY Nobel Lecture, December 8, 2002 by RICCARDO GIACCONI Associated Universities, Inc., Suite 730, 1400 16th St., NW, Washington, DC 20036, USA, and Johns Hopkins University, 3400 N. Charles Street, Baltimore, USA. 1.0 INTRODUCTION The development of rockets and satellites capable of carrying instruments outside the absorbing layers of the Earth’s atmosphere has made possible the observation of celestial objects in the x-ray range of wavelength. X-rays of energy greater than several hundreds of electron volts can pene- trate the interstellar gas over distances comparable to the size of our own galaxy, with greater or lesser absorption depending on the direction of the line of sight. At energies of a few kilovolts, x-rays can penetrate the entire col- umn of galactic gas and in fact can reach us from distances comparable to the radius of the universe. The possibility of studying celestial objects in x-rays has had a profound sig- nificance for all astronomy. Over the x-ray to gamma-ray range of energies, x- rays are, by number of photons, the most abundant flux of radiation that can reveal to us the existence of high energy events in the cosmos. By high ener- gy events I mean events in which the total energy expended is extremely high (supernova explosions, emissions by active galactic nuclei, etc.) or in which the energy acquired per nucleon or the temperature of the matter involved is extremely high (infall onto collapsed objects, high temperature plasmas, in- teraction of relativistic electrons with magnetic or photon fields). -
Simulated Observations of Galaxy Clusters for Current and Future X-Ray Surveys
TECHNISCHE UNIVERSITAT¨ MUNCHEN¨ MAX-PLANCK-INSTITUT FUR¨ EXTRATERRESTRISCHE PHYSIK Simulated Observations of Galaxy Clusters for Current and Future X-ray Surveys Martin L. Muhlegger¨ Vollst¨andiger Abdruck der von der Fakult¨at f¨ur Physik der Technischen Universit¨at M¨unchen zur Erlangung des akademischen Grades eines Doktors der Naturwissenschaften (Dr. rer. nat.) genehmigten Dissertation. Vorsitzender: Univ.-Prof. Dr. M. Ratz Pr¨ufer der Dissertation: 1. Hon.-Prof. Dr. G. Hasinger 2. Univ.-Prof. Dr. L. Oberauer Die Dissertation wurde am 27.07.2010 bei der Technischen Universit¨at M¨unchen eingereicht und durch die Fakult¨at f¨ur Physik am 02.11.2010 angenommen. TECHNISCHE UNIVERSITAT¨ MUNCHEN¨ Simulated Observations of Galaxy Clusters for Current and Future X-ray Surveys Dissertation von Martin L. Muhlegger¨ 10. November 2010 MAX-PLANCK-INSTITUT FUR¨ EXTRATERRESTRISCHE PHYSIK iv Abstract Clusters of galaxies are versatile tools for both astrophysics and cosmology. In the X-ray band, clusters are clearly identifiable by their distinct extended emission from the hot Intra Cluster Medium, which qualifies X-ray search techniques as a preferred method for cluster surveys. One of the currently active X-ray cluster surveys is the XMM-Newton Distant Cluster Project (XDCP), with the main objective to identify and study high redshift (z & 0.8) clusters using XMM archival data. The next generation all-sky X-ray cluster survey will be performed with eROSITA (extended ROentgen Survey with an Imaging Telescope Array), which is currently under development in a Russian-German collaboration led by the Max-Planck-Institute for Extraterrestrial Physics (MPE). Identifying clusters in X-ray surveys is a challenging task, but has the advantage that the surveys can be calibrated by simulations to fully exploit them in statistical studies.