Model Analysis of the Pathfinder Boiling Water Reactor Francis Vito Rossano Iowa State University
Total Page:16
File Type:pdf, Size:1020Kb
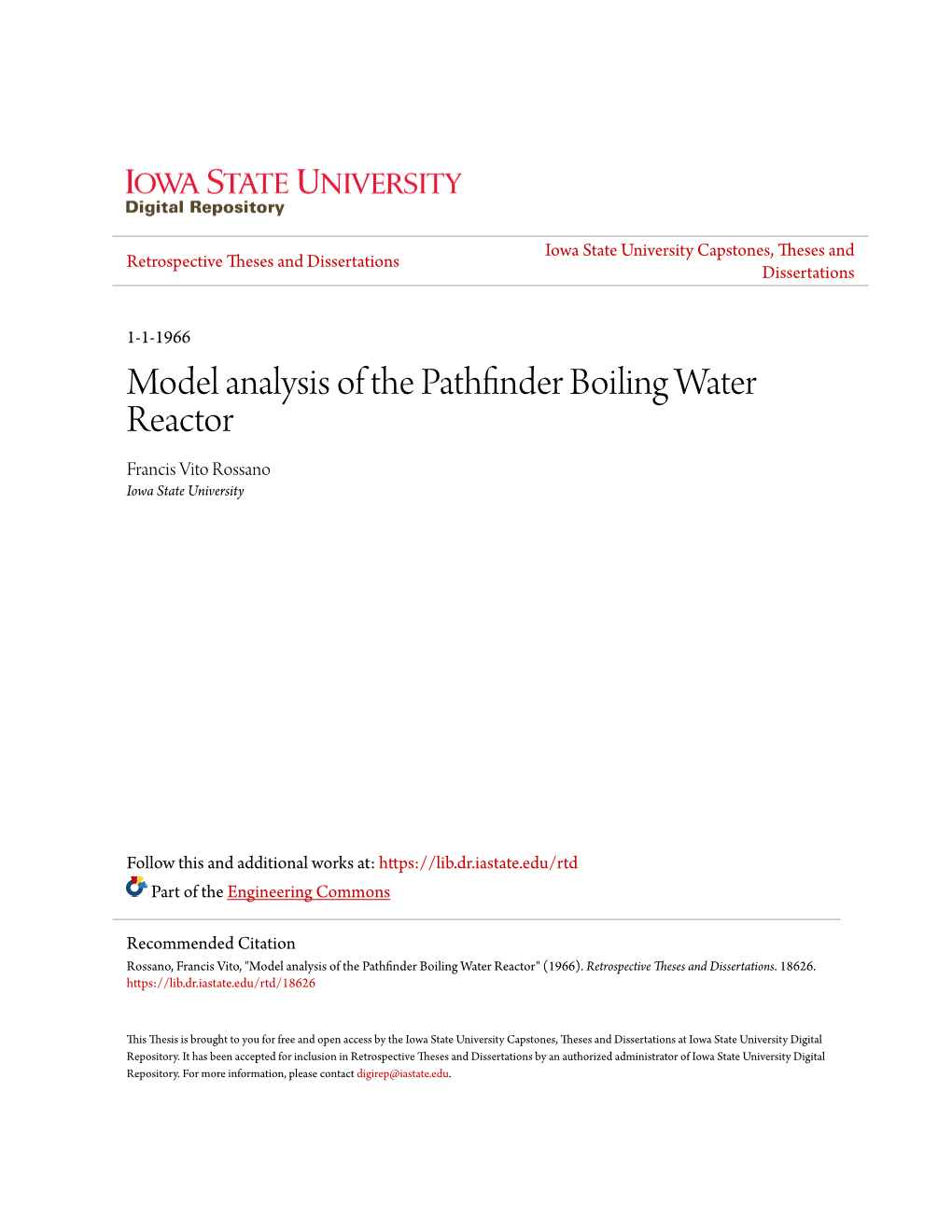
Load more
Recommended publications
-
Model and Prototype Unit E-1: List of Subjects
ES206 Fluid Mechanics UNIT E: Model and Prototype ROAD MAP . E-1: Dimensional Analysis E-2: Modeling and Similitude ES206 Fluid Mechanics Unit E-1: List of Subjects Dimensional Analysis Buckingham Pi Theorem Common Dimensionless Group Experimental Data Page 1 of 9 Unit E-1 Dimensional Analysis (1) p = f (D, , ,V ) SLIDE 1 UNIT F-1 DimensionalDimensional AnalysisAnalysis (1)(1) ➢ To illustrate a typical fluid mechanics problem in which experimentation is required, consider the steady flow of an incompressible Newtonian fluid through a long, smooth-walled, horizontal, circular pipe: ➢ The pressure drop per unit length ( p ) is a function of: ➢ Pipe diameter (D ) SLIDE 2➢ Fluid density ( ) UNIT F-1 ➢ Fluid viscousity ( ) ➢ Mean velocity ( V ) p = f (D, , ,V ) DimensionalDimensional AnalysisAnalysis (2)(2) ➢ To perform experiments, it would be necessaryTextbook (Munson, Young, to and change Okiishi), page 403 one of the variables while holding others constant ??? How can we combine these data to obtain the desired general functional relationship between pressure drop and variables ? Textbook (Munson, Young, and Okiishi), page 347 Page 2 of 9 Unit E-1 Dimensional Analysis (2) Dp VD = 2 V SLIDE 3 UNIT F-1 DimensionalDimensional AnalysisAnalysis (3)(3) ➢ Fortunately, there is a much simpler approach to this problem that will eliminate the difficulties ➢ It is possible to collect variables and combine into two non-dimensional variables (dimensionless products or groups): Dp VD = 2 V SLIDE 4 UNIT F-1 Pressure Reynolds -
On Dimensionless Numbers
chemical engineering research and design 8 6 (2008) 835–868 Contents lists available at ScienceDirect Chemical Engineering Research and Design journal homepage: www.elsevier.com/locate/cherd Review On dimensionless numbers M.C. Ruzicka ∗ Department of Multiphase Reactors, Institute of Chemical Process Fundamentals, Czech Academy of Sciences, Rozvojova 135, 16502 Prague, Czech Republic This contribution is dedicated to Kamil Admiral´ Wichterle, a professor of chemical engineering, who admitted to feel a bit lost in the jungle of the dimensionless numbers, in our seminar at “Za Plıhalovic´ ohradou” abstract The goal is to provide a little review on dimensionless numbers, commonly encountered in chemical engineering. Both their sources are considered: dimensional analysis and scaling of governing equations with boundary con- ditions. The numbers produced by scaling of equation are presented for transport of momentum, heat and mass. Momentum transport is considered in both single-phase and multi-phase flows. The numbers obtained are assigned the physical meaning, and their mutual relations are highlighted. Certain drawbacks of building correlations based on dimensionless numbers are pointed out. © 2008 The Institution of Chemical Engineers. Published by Elsevier B.V. All rights reserved. Keywords: Dimensionless numbers; Dimensional analysis; Scaling of equations; Scaling of boundary conditions; Single-phase flow; Multi-phase flow; Correlations Contents 1. Introduction ................................................................................................................. -
Module 6 : Lecture 1 DIMENSIONAL ANALYSIS (Part – I) Overview
NPTEL – Mechanical – Principle of Fluid Dynamics Module 6 : Lecture 1 DIMENSIONAL ANALYSIS (Part – I) Overview Many practical flow problems of different nature can be solved by using equations and analytical procedures, as discussed in the previous modules. However, solutions of some real flow problems depend heavily on experimental data and the refinements in the analysis are made, based on the measurements. Sometimes, the experimental work in the laboratory is not only time-consuming, but also expensive. So, the dimensional analysis is an important tool that helps in correlating analytical results with experimental data for such unknown flow problems. Also, some dimensionless parameters and scaling laws can be framed in order to predict the prototype behavior from the measurements on the model. The important terms used in this module may be defined as below; Dimensional Analysis: The systematic procedure of identifying the variables in a physical phenomena and correlating them to form a set of dimensionless group is known as dimensional analysis. Dimensional Homogeneity: If an equation truly expresses a proper relationship among variables in a physical process, then it will be dimensionally homogeneous. The equations are correct for any system of units and consequently each group of terms in the equation must have the same dimensional representation. This is also known as the law of dimensional homogeneity. Dimensional variables: These are the quantities, which actually vary during a given case and can be plotted against each other. Dimensional constants: These are normally held constant during a given run. But, they may vary from case to case. Pure constants: They have no dimensions, but, while performing the mathematical manipulation, they can arise. -
The Dimnum Package
The dimnum package Miguel R. Clemente [email protected] v1.0.1 from 2021/04/05 Note: Prandtl number is redefined from the amsmath package. 1 Introduction This package simplifies the calling of Dimensionless Numbers in math or text mode. In Table 1 you can find all available Dimensionless Numbers. 2 Usage A Dimensionless number is composed of four items: the command, the symbol, the name, its identifier. You can call a Dimensionless Number in three distinct ways: by its symbol { using the command (i.e. \Ar { Ar). by its name (short version) { appending [s] to the command (i.e. \Bi[s] { Biot). by its name and identifier (long version) { appending [l] to the command (i.e. \Kn[l] { Knudsen number). Symbol, short and long versions, all work in math or text mode without the need of further commands. 1 Besides the comprehensive list of included Dimensionless Numbers, this pack- age also introduces a command to create new Dimensionless Numbers. Creating a Dimensionless Number is achieved by using \newdimnum{\command}{symbol}{name}{identifier} for example, to add the Morton number we write \newdimnum{\Mo}{Mo}{Morton}{number} The identifier can be left empty, such as in the case of Drag Coefficient \newdimnum{Cd}{\ensuremath{C_d}}{Drag Coefficient}{} in this example we also introduce an important command. When the Dimension- less Number symbol is always expressed in math mode { either by definition or the use of subscripts or superscripts { we add \ensuremath{} to encompass the symbol, ensuring a proper representation of the Dimensionless Number. You can add your own Dimensionless Numbers to your projects. -
SASO-ISO-80000-11-2020-E.Pdf
SASO ISO 80000-11:2020 ISO 80000-11:2019 Quantities and units - Part 11: Characteristic numbers ICS 01.060 Saudi Standards, Metrology and Quality Org (SASO) ----------------------------------------------------------------------------------------------------------- this document is a draft saudi standard circulated for comment. it is, therefore subject to change and may not be referred to as a saudi standard until approved by the boardDRAFT of directors. Foreword The Saudi Standards ,Metrology and Quality Organization (SASO)has adopted the International standard No. ISO 80000-11:2019 “Quantities and units — Part 11: Characteristic numbers” issued by (ISO). The text of this international standard has been translated into Arabic so as to be approved as a Saudi standard. DRAFT DRAFT SAUDI STANDADR SASO ISO 80000-11: 2020 Introduction Characteristic numbers are physical quantities of unit one, although commonly and erroneously called “dimensionless” quantities. They are used in the studies of natural and technical processes, and (can) present information about the behaviour of the process, or reveal similarities between different processes. Characteristic numbers often are described as ratios of forces in equilibrium; in some cases, however, they are ratios of energy or work, although noted as forces in the literature; sometimes they are the ratio of characteristic times. Characteristic numbers can be defined by the same equation but carry different names if they are concerned with different kinds of processes. Characteristic numbers can be expressed as products or fractions of other characteristic numbers if these are valid for the same kind of process. So, the clauses in this document are arranged according to some groups of processes. As the amount of characteristic numbers is tremendous, and their use in technology and science is not uniform, only a small amount of them is given in this document, where their inclusion depends on their common use. -
7 Similitude, Dimensional Analysis, and Modeling
7708d_c07_384* 7/23/01 10:00 AM Page 384 Flow past a circular cylinder with Re ϭ 2000: The pathlines of flow past any circular cylinder regardless of size, velocity, or fluid are as shown provided that the dimensionless1 parameter called the Reynolds2 number, Re, is equal to 2000. For other values of Re, the flow pattern will be different air bubbles in water . Photograph courtesy of ONERA, France. 1 2 1 2 7708d_c07_384* 7/23/01 10:00 AM Page 385 7 Similitude, Dimensional Analysis, and Modeling Although many practical engineering problems involving fluid mechanics can be solved by using the equations and analytical procedures described in the preceding chapters, there re- main a large number of problems that rely on experimentally obtained data for their solu- tion. In fact, it is probably fair to say that very few problems involving real fluids can be Experimentation solved by analysis alone. The solution to many problems is achieved through the use of a and modeling are combination of analysis and experimental data. Thus, engineers working on fluid mechanics widely used tech- problems should be familiar with the experimental approach to these problems so that they niques in fluid can interpret and make use of data obtained by others, such as might appear in handbooks, mechanics. or be able to plan and execute the necessary experiments in their own laboratories. In this chapter we consider some techniques and ideas that are important in the planning and exe- cution of experiments, as well as in understanding and correlating data that may have been obtained by other experimenters. -
River Hydraulics
River Hydraulics GEOLOGICAL SURVEY WATER-SUPPLY PAPER 1369 This water-supply paper was published as separate chapters A-E UNITED STATES DEPARTMENT OF THE INTERIOR STEWART L. UDALL, Secretary GEOLOGICAL SURVEY Thomas B. Nolan, Director CONTENTS [The letters in parentheses designate separately published chapters] Page (A) Selected topics of fluid mechanics, by Carl E. Kindsvater_______ 1 (B) Energy losses associated with abrupt enlargements in pipes, with special reference to the influence of boundary roughness, by Carl E. Kindsvater ___________________________________ 53 (C) Surges in natural stream channels, by S. B. Rantz ___ 77 (D) Flow through openings in width constrictions, by Jacob Davidian, P. H. Carrigan, Jr., and John Shen____________________ 91 (E) Stream gaging control structure for the Rio Grande conveyance chan nel near Bernardo, New Mexico, by D. D. Harris and E. V. Richardson ___________________________________ 123 O Selected Topics Of Fluid Mechanics GEOLOGICAL SURVEY WATER-SUPPLY PAPER 1369-A Selected Topics Of Fluid Mechanics By CARL E. KINDSVATER RIVER HYDRAULICS GEOLOGICAL SURVEY WATER-SUPPLY PAPER 1369-A A discussion of the concepts of fluid mechanics which are the foundations of river hydraulics UNITED STATES GOVERNMENT PRINTING OFFICE, WASHINGTON : 1958 UNITED STATES DEPARTMENT OF THE INTERIOR FRED A. SEATON, Secretary GEOLOGICAL SURVEY Thomas B. Nolan, Director For sale by the Superintendent of Documents, U. S. Government Printing Office Washington 25, D. C. - Price 55 cents CONTENTS Page Abstract- ___________________________________...._-___------------__. -
Engineering Fluid Mechanics
T. Al-Shemmeri Engineering Fluid Mechanics Download free eBooks at bookboon.com 2 Engineering Fluid Mechanics © 2012 T. Al-Shemmeri & Ventus Publishing ApS ISBN 978-87-403-0114-4 Download free eBooks at bookboon.com 3 Engineering Fluid Mechanics Contents Contents Notation 7 1 Fluid Statics 14 1.1 Fluid Properties 14 1.2 Pascal’s Law 21 1.3 Fluid-Static Law 21 1.4 Pressure Measurement 24 1.5 Centre of pressure & the Metacentre 29 1.6 Resultant Force and Centre of Pressure on a Curved Surface in a Static Fluid 34 1.7 Buoyancy 37 1.8 Stability of floating bodies 40 1.9 Tutorial problems 45 2 Internal Fluid Flow 47 2.1 Definitions 47 2.2 Conservation of Mass 50 2.3 Conservation of Energy 52 2.4 Flow Measurement 54 2.5 Flow Regimes 58 The next step for top-performing graduates Masters in Management Designed for high-achieving graduates across all disciplines, London Business School’s Masters in Management provides specific and tangible foundations for a successful career in business. This 12-month, full-time programme is a business qualification with impact. In 2010, our MiM employment rate was 95% within 3 months of graduation*; the majority of graduates choosing to work in consulting or financial services. As well as a renowned qualification from a world-class business school, you also gain access to the School’s network of more than 34,000 global alumni – a community that offers support and opportunities throughout your career. For more information visit www.london.edu/mm, email [email protected] or give us a call on +44 (0)20 7000 7573. -
2002 05 Qualification of Ultrasonic Flow Meters for Custody Transfer of Natural Gas Hill GE Panametrics
Paper 2.1 Qualification of Ultrasonic Flow Meters for Custody Transfer of Natural Gas Using Atmospheric Air Calibration Facilities Jim Hill, GE Panametrics Andreas Weber, RMG Messtechnik Joern Weber, RMG Messtechnik North Sea Flow Measurement Workshop 22nd – 25th October 2002 Qualification of Ultrasonic Flow Meters for Custody Transfer of Natural Gas Using Atmospheric Air Calibration Facilities Jim Hill, GE Panametrics Andreas Weber, RMG Messtechnik Joern Weber, RMG Messtechnik 1 INTRODUCTION The ability to operate in atmospheric air was a significant factor in the joint development by GE Panametrics and RMG Messtechnik of a custody transfer meter. Using air as a test medium yields major benefits. Two benefits are cost and availability. Natural gas calibration facilities cost thousands of dollars per day to use. This expense alone can accumulate into millions of dollars over the development of a meter, forming a barrier to entry to meter manufacturers. Also, the flow rate, if any, available at a natural gas test facility is dependent on seasonal, and even daily demand. The another factor is safety. Working at atmospheric pressure allows the developer to quickly make prototypes and design, without the safety concerns involved with high-pressure gas. Also, tests can be conducted with electronics exposed so that the performance of individual circuits and embedded software can be monitored, without the risk of encountering combustible gas. The final advantage is the flexibility that an open-loop calibration facility provides. Since the loop draws air from an open room, almost infinite combinations of installation conditions can be created. This is important, as actual field installations are a continuous distribution of configurations. -
Copyrighted Material
BBMIndx.inddMIndx.indd PPageage I-1I-1 111/10/181/10/18 6:536:53 PMPM f-389f-389 //208/WB02304_EVALCS/9781119469582/bmmatter/text_s208/WB02304_EVALCS/9781119469582/bmmatter/text_s Index Absolute pressure, 13, 41 Angular momentum flow, 174, video V5.12 restrictions on use of, 106–110, 222, Absolute temperature Annulus, flow in, 257–258 video V3.12 in British Gravitational (BG) System, 7 Apparent viscosity, 16 unsteady flow, 107–108 in English Engineering (EE) System, 8 Aqueducts, 454 use of, video V3.2 in ideal gas law, 12 Archimedes, 27, 28, 62 Best efficiency points, 564 in International System (SI), 7 Archimedes’ principle, 61–64, video V2.7 Best hydraulic cross section, 460–461 Absolute velocity Area BG units. see British Gravitational (BG) System in centrifugal pumps, 558–561 centroid, 51–53 of units defined, 154, 551 compressible flow with change in, 507–522 Bingham plastic, 16–17 in rotating sprinkler example, 176–177 isentropic flow of ideal gas with change in, Birds, wings of, 429 in turbo machine example, 551–554 510–516 Blades vs. relative velocity, 141–142 and Mach number, 511–513 curved and radia, 561, 562 Absolute viscosity moment of inertia, 52 radial, 561 of air, 620–621 Aspect ratio rotor, 575, 591 of common gases, 617 air foil, 435 stator, 575, 591 of common liquids, 617 plate, 271 turbo machine, 549 defined, 15 Assemblies, 603 Blade velocity, 551–554, 558–559 of U.S. Standard Atmosphere, 622–623 Atmosphere, standard. see Standard Blasius, H., 29, 285, 389 of water, 619 atmosphere Blasius formula, 285, 339 Acceleration -
Mechanics of a Plant in Fluid Flow Highlight Keywords Abstract
Posted online 05/16/2019 Mechanics of a Plant in Fluid Flow F.P. Gosselin Mechanics of a Plant in Fluid Flow Frédérick P. Gosselin Laboratory for Multiscale Mechanics, Department of Mechanical Engineering, Polytechnique Montréal, Montréal, QC, Canada Department of Botany, University of British Columbia, Vancouver, BC, Canada [email protected] Highlight Plants reduce their drag by deforming in the flow, sway with the waves and make use of flow-induced vibrations to increase their exchange with the fluid they live in. Keywords Aerodynamics; Current; Drag; Elasticity; Flow-Induced Vibrations; Fluid-Structure Interactions; Hydrodynamics; Loads; Pollen Release and Capture; Reconfiguration; Waves; Wind Abstract Plants live in constantly moving fluid, whether air or water. In response to the loads associated with fluid motion, plants bend and twist, often with great amplitude. These large deformations are not found in traditional engineering application and thus necessitate new specialised scientific developments. Studying Fluid-Structure Interactions (FSI) in botany, forestry and agricultural science is crucial to the optimisation of biomass production for food, energy, and construction materials. FSI are also central in the study of the ecological adaptation of plants to their environment. This review paper surveys the mechanics of FSI on individual plants. We present a short refresher on fluids mechanics then dive in the statics and dynamics of plant-fluid interactions. For every phenomenon considered, we present the appropriate dimensionless numbers to characterise the problem, discuss the implications of these phenomena on biological processes, and propose future research avenues. We cover the concept of reconfiguration while considering poroelasticity, torsion, chirality, buoyancy, and skin friction. -
Dimensionless Numbers
Dimensionless Numbers https://www.iist.ac.in/sites/default/files/people/numbers.html Dimensionless Numbers A. Salih Dept. of Aerospace Engineering IIST, Thiruvananthapuram The nondimensionalization of the governing equations of fluid flow is important for both theoretical and computational reasons. Nondimensional scaling provides a method for developing dimensionless groups that can provide physical insight into the importance of various terms in the system of governing equations. Computationally, dimensionless forms have the added benefit of providing numerical scaling of the system discrete equations, thus providing a physically linked technique for improving the ill-conditioning of the system of equations. Moreover, dimensionless forms also allow us to present the solution in a compact way. Some of the important dimensionless numbers used in fluid mechanics and heat transfer are given below. Nomenclature Archimedes Number: 3 Re 2 gL ρ(ρs − ρ) Ar = = Fr µ2 Atwood Number: (ρ1 − ρ2) A = (ρ1 + ρ2) Note: Used in the study of density stratified flows. Biot Number: hL conductive resistance in solid Bi = = Ks convective resistance in thermal boundary layer Bond Number: We ρgL 2 Bo = = Fr σ Brinkman Number: µU2 Br = K(Tw − T o) Note: Brinkman number is related to heat conduction from a wall to a flowing viscous fluid. It is commonly used in polymer processing. Capillary Number: We Uµ Ca = = Re σ Cauchy Number: ρU2 Ca = M2 = K 1 06.10.2017, 09:49 Dimensionless Numbers https://www.iist.ac.in/sites/default/files/people/numbers.html Centrifuge Number: We ρΩ 2L3 Ce = = Ro 2 σ Dean Number: Re De = (R ⁄ h )1/2 Note: Dean number deals with the stability of two- dimensional flows in a curved channel with mean radius R and width 2h .