Soil Phosphorus Forms Along a Strong Nutrient Gradient in a Tropical Ombrotrophic Wetland
Total Page:16
File Type:pdf, Size:1020Kb
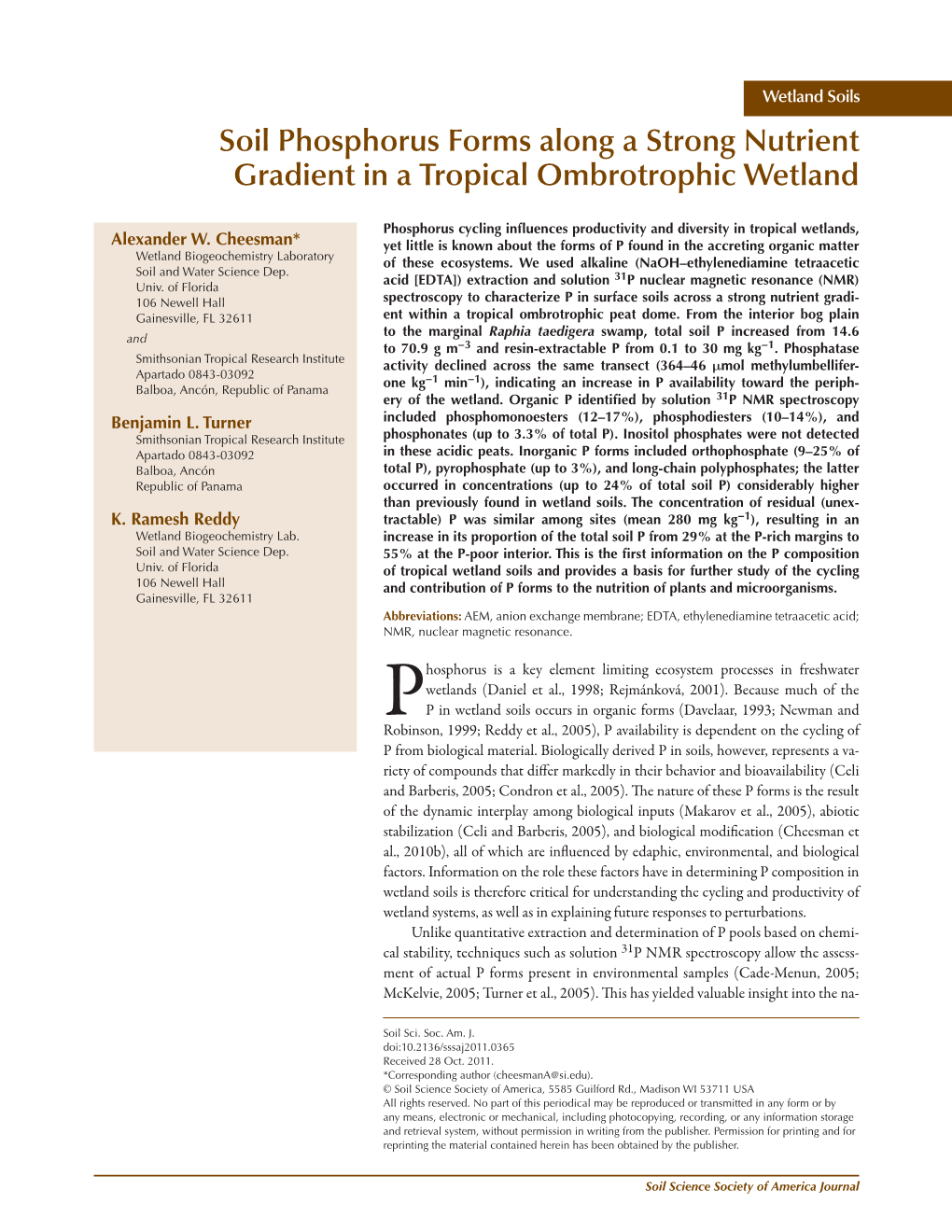
Load more
Recommended publications
-
The Structure and Composition of Vegetation in the Lake-Fill Peatlands of Indiana
2001. Proceedings of the Indiana Academy of Science 1 10:51-78 THE STRUCTURE AND COMPOSITION OF VEGETATION IN THE LAKE-FILL PEATLANDS OF INDIANA Anthony L. Swinehart 1 and George R. Parker: Department of Forestry and Natural Resources, Purdue University, West Lafayette, Indiana 47907 Daniel E. Wujek: Department of Biology, Central Michigan University, Mount Pleasant, Michigan 48859 ABSTRACT. The vegetation of 16 lake-fill peatlands in northern Indiana was systematically sampled. Peatland types included fens, tall shrub bogs, leatherleaf bogs and forested peatlands. No significant difference in species richness among the four peatland types was identified from the systematic sampling. Vegetation composition and structure, along with water chemistry variables, was analyzed using multi- variate statistical analysis. Alkalinity and woody plant cover accounted for much of the variability in the herbaceous and ground layers of the peatlands, and a successional gradient separating the peatlands was evident. A multivariate statistical comparison of leatherleaf bogs from Indiana, Michigan, Ohio, New York, New Jersey and New Hampshire was made on the basis of vegetation composition and frequency and five climatic variables. The vascular vegetation communities of Indiana peatlands and other peatlands in the southern Great Lakes region are distinct from those in the northeastern U.S., Ohio and the northern Great Lakes. Some of these distinctions are attributed to climatic factors, while others are related to biogeo- graphic history of the respective regions. Keywords: Peatlands, leatherleaf bogs, fens, ecological succession, phytogeography Within midwestern North America, the such as Chamaedaphne calyculata, Androm- northern counties of Illinois, Indiana and Ohio eda glaucophylla, and Carex oligospermia of- 1 represent the southern extent of peatland com- ten make "southern outlier peatlands ' con- munities containing characteristic plant spe- spicuous to botanists, studies of such cies of northern or boreal affinity. -
Does Soil Fertility Influence the Vegetation Diversity of a Tropical Peat Swamp
Does soil fertility influence the vegetation diversity of a tropical peat swamp forest in Central Kalimantan, Indonesia? By Leanne Elizabeth Milner Dissertation presented for the Honours degree of BSc Geography Department of Geography University of Leicester 24 th February 2009 Approx number of words (12,000) 1 Contents Page LIST OF FIGURES I LIST OF TABLES II ABSTRACT III ACKNOWLEGEMENTS IV Chapter 1: Introduction 1 1.1 Aim 2 1.2 Objectives 2 1.3 Hypotheses 2 1.4 Scientific Background and Justification 3 1.5 Literature Review 7 1.5.1 Soil Fertility and Vegetation Species Diversity 7 1.5.2 Tropical Peatlands 7 1.5.3 Vegetation and Soil in tropical peatlands 8 1.5.4 Hydrology 14 1.5.5 Phenology and Rainfall 15 Chapter 2 : Methodology 17 2.1 Study Site and Transects 18 2.2 Soil Analysis 21 2.3 Chemical Analysis 22 2.4 Tree Data 25 2.5 Phenology Data 25 2.6 Rainfall Data 26 2.7 Data Analysis 26 2.7.1 Soil Data Analysis 26 2.7.2 Tree Data Analysis 26 2.7.3 Phenology Data Analysis 28 2 2.7.4 Rainfall Data Analysis 28 Chapter 3: Analysis 29 3.1 Tree and Liana Analysis 30 3.1.1 Basal Area and Density 31 3.1.2 Relative Importance Values 33 3.2 Peat Chemistry Analysis 35 3.3 Tree Phenology Analysis 43 3.4 Rainfall Analysis 46 Chapter 4: Discussion 47 4.1 Overall Findings 48 4.2 Peat Chemistry 48 4.3 Vegetation and Phenology 51 4.4 Peat Depth and Gradient 53 4.5 Significance of the Water Table 54 4.6 Limitations and Areas for further Research 56 Chapter 5: Conclusion 59 5.0 Conclusion 60 REFERENCES 62 APPENDICES 67 Appendix A: Soil Nutrient Analysis 68 Appendix B: Regression Outputs 70 Appendix C: Tree Data ON CD Appendix D: Phenology Data ON CD 3 List of Figures Figure 1 – Distribution of tropical peatlands in South East Asia and location of the study area. -
CHEMICAL and PHYSICAL CHARACTERISTICS of SHALLOW GROUND WATERS in NORTHERN MICHIGAN BOGS, SWAMPS, and Fensl
Amer. J. Bot. 69(8): 1231-1239. 1982. CHEMICAL AND PHYSICAL CHARACTERISTICS OF SHALLOW GROUND WATERS IN NORTHERN MICHIGAN BOGS, SWAMPS, AND FENSl 2 4 CHRISTA R. SCHWINTZER ,3 AND THOMAS J. TOMBERLIN The University of Michigan Biological Station," Pellston, Michigan 49769, Harvard University, Harvard Forest, Petersham, Massachusetts 01366, and Department of Statistics! Harvard University, Cambridge, Massachusetts 02138 ABSTRACT Fifteen chemical and physical characteristics were examined in samples of shallow ground water taken in midsummerat 15-30em below the surface in six bogs, 15 swamps, and six fens. The wetland types were identified on the basis of their vegetation. Three groups of covarying water characteristics were identified by factor analysis. Factor I included Ca, Mg, Si, pH, alkalinity, conductivity and to a much lesser extent Na, and reflects the degree of telluric water influence in the wetland. Factor 2 included reactive-P, total-P, NH,,-N, and to a lesser extent K, and consists of elements that primarily enter interstitial water via organic matter decom position. Factor 3 included Na, Cl, and to a much lesser extent K. The wetlands formed two distinct groups with respect to water chemistry: weakly minero trophic (pH 3.8-4.3) including all bogs and moderately to strongly minerotrophic (pH 5.5-7.4) includingall swamps and fens. The bogs had very low values for Factor 1 characteristics and moderate values for the remaining characteristics. The swamps and fens had moderate to high values for Factor I characteristics and showed considerable overlap in this respect. The fens had consistently low values for Factor 2 characteristics but overlapped with some swamps which also had low Factor 2 scores. -
Wetland and Hydric Soils 6 Carl C
Wetland and Hydric Soils 6 Carl C. Trettin, Randall K. Kolka, Anne S. Marsh, Sheel Bansal, Erik A. Lilleskov, Patrick Megonigal, Marla J. Stelk, Graeme Lockaby, David V. D’Amore, Richard A. MacKenzie, Brian Tangen, Rodney Chimner, and James Gries Introduction conterminous United States have been converted to other land uses over the past 150 years (Dahl 1990). Most of those losses Soil and the inherent biogeochemical processes in wetlands are due to draining and conversion to agriculture. States in the contrast starkly with those in upland forests and rangelands. The Midwest such as Iowa, Illinois, Missouri, Ohio, and Indiana differences stem from extended periods of anoxia, or the lack of have lost more than 85% of their original wetlands, and oxygen in the soil, that characterize wetland soils; in contrast, California has lost 96% of its wetlands. In the mid-1900s, the upland soils are nearly always oxic. As a result, wetland soil importance of wetlands in the landscape started to be under- biogeochemistry is characterized by anaerobic processes, and stood, and wetlands are now recognized for their inherent wetland vegetation exhibits specifc adaptations to grow under value that is realized through the myriad of ecosystem ser- these conditions. However, many wetlands may also have peri- vices, including storage of water to mitigate fooding, fltering ods during the year where the soils are unsaturated and aerated. water of pollutants and sediment, storing and sequestering car- This fuctuation between aerated and nonaerated soil condi- bon (C), providing critical habitat for wildlife, and recreation. tions, along with the specialized vegetation, gives rise to a wide Historically, drainage of wetlands for agricultural devel- variety of highly valued ecosystem services. -
Washington Natural Heritage Program Presented to the Natural Heritage Advisory Council December 5, 2014
Crowberry Bog Proposed Natural Area Preserve Natural Area Recommendation Submitted by Washington Natural Heritage Program Presented to the Natural Heritage Advisory Council December 5, 2014 Size Three boundary options are presented in this document ranging in size from 248 to 469 acres. Option 1 is 258 acres, Option 2 is 348 acres and Option 3 is 469 acres. Location The site is located within the Pacific Coast ecoregion, approximately 2.5 miles east of Highway 101, 0.3 miles south of the Hoh River and 0.3 miles north of the Hoh Mainline Road in Jefferson County (Figures 1 and 2). The legal description for each option proposed boundary is: Option 1(Figure 3): portions of T27N R12W Sec 25; Sec 26; Sec. 35, and Sec 36. Option 2 (Figure 4): portions of T27N R11W Sec 31; T27N R12W Sec 25; Sec 26; Sec 35, Sec 36; T27N R11W Sec. 31. Option 3 (Figure 5): portions of T27N R11W Sec. 31; T27N R12W Sec 25; Sec 26; Sec 35; and Sec 36. Ownership Ownership is primarily Washington Department of Natural Resources (DNR) with the remainder including DNR-held conservation easements on private lands, and Fruit Growers Supply Co. (Figure 6). Primary Features Crowberry Bog may be the only known coastal plateau bog in the western conterminous United States and the southern-most known in western North America. The proposed NAP (pNAP) would provide an opportunity to protect this unique and very rare feature as well as three elements listed as priorities in the 2011 State of Washington Natural Heritage Plan (Table 1; Figures 3-5): Forested Sphagnum Bog (Priority 2), Low Elevation Sphagnum Bog (Priority 3), and Makah copper butterfly (Priority 2). -
773593683.Pdf
LAKE AREA CHANGE IN ALASKAN NATIONAL WILDLIFE REFUGES: MAGNITUDE, MECHANISMS, AND HETEROGENEITY A DISSERTATION Presented to the Faculty of the University of Alaska Fairbanks In Partial Fulfillment of the Requirements for the Degree of DOCTOR OF PHILOSOPHY By Jennifer Roach, B.S. Fairbanks, Alaska December 2011 iii Abstract The objective of this dissertation was to estimate the magnitude and mechanisms of lake area change in Alaskan National Wildlife Refuges. An efficient and objective approach to classifying lake area from Landsat imagery was developed, tested, and used to estimate lake area trends at multiple spatial and temporal scales for ~23,000 lakes in ten study areas. Seven study areas had long-term declines in lake area and five study areas had recent declines. The mean rate of change across study areas was -1.07% per year for the long-term records and -0.80% per year for the recent records. The presence of net declines in lake area suggests that, while there was substantial among-lake heterogeneity in trends at scales of 3-22 km a dynamic equilibrium in lake area may not be present. Net declines in lake area are consistent with increases in length of the unfrozen season, evapotranspiration, and vegetation expansion. A field comparison of paired decreasing and non-decreasing lakes identified terrestrialization (i.e., expansion of floating mats into open water with a potential trajectory towards peatland development) as the mechanism for lake area reduction in shallow lakes and thermokarst as the mechanism for non-decreasing lake area in deeper lakes. Consistent with this, study areas with non-decreasing trends tended to be associated with fine-grained soils that tend to be more susceptible to thermokarst due to their higher ice content and a larger percentage of lakes in zones with thermokarst features compared to study areas with decreasing trends. -
Great Sidney Bog Beginning with Focus Areas of Statewide Ecological Significance Habitat Great Sidney Bog
Focus Areas of Statewide Ecological Significance: Great Sidney Bog Beginning with Focus Areas of Statewide Ecological Significance Habitat Great Sidney Bog Biophysical Region • Central Maine Embayment WHY IS THIS AREA SIGNIFICANT? Rare and Exemplary Because of its size, vegetation, and relatively undisturbed Natural Communities nature, the Great Sidney Bog is considered a very good Raised Level Bog Ecosystem example of a Raised Level Bog Ecosystem. Its ecological importance is augmented by its location in the southern Significant Wildlife Habitats Inland Wading Bird and Waterfowl third of the state, where raised bogs become much Deer Wintering Area scarcer than they are further north. The easy access to this peatland in the Waterville – Augusta area enhances its value for education and research. OPPORTUNITIES FOR CONSERVATION » Educate recreational users about the ecological and economic benefits provided by the focus area. » Encourage best management practices for forestry, vegetation clearing, and soil disturbance activities near significant features. » Maintain intact forested buffers along water bodies and wetlands. » Work with landowners to encourage sustainable for- est management and mining practices on remaining privately owned forest lands. » Work with willing landowners to permanently protect undeveloped areas and significant features. For more conservation opportunities, visit the Beginning with Habitat Online Toolbox: www.beginningwithhabitat. org/toolbox/about_toolbox.html. Photo credits, top to bottom: Maine Natural Areas Program, Lisa St. Hilaire (photos 4-5) 1 Focus Areas of Statewide Ecological Significance: Great Sidney Bog Great Sidney Bog, Maine Natural Areas Program FOCUS AREA OVERVIEW Great Sidney Bog is a 605-acre Raised Level Bog lying between parts of level bogs are somewhat raised (though not domed), the Belgrade Lakes chain and the Kennebec River. -
Ecohydrological Characteristics of a Newly Identified Coastal Raised Bog on the Western Olympic Peninsula, Washington State, USA
Received: 3 September 2020 Revised: 12 December 2020 Accepted: 9 February 2021 DOI: 10.1002/eco.2287 RESEARCH ARTICLE Ecohydrological characteristics of a newly identified coastal raised bog on the western Olympic Peninsula, Washington State, USA F. Joseph Rocchio1 | Edward Gage2 | Tynan Ramm-Granberg1 | Andrea K. Borkenhagen2 | David J. Cooper2 1Washington Department of Natural Resources, Natural Heritage Program, Abstract Olympia, Washington, USA In western North America, ombrotrophic bogs are known to occur as far south as 2 Department of Forest and Rangeland coastal regions of British Columbia. A recent discovery of a peatland with a raised Stewardship, Colorado State University, Fort Collins, Colorado, USA peat surface on the western Olympic Peninsula in Washington State (Crowberry Bog), USA, suggested that the distribution range of this ecosystem type extends fur- Correspondence F. Joseph Rocchio, Washington Department of ther south along the coast. To confirm if the site was an ombrotrophic peatland, we Natural Resources, Natural Heritage Program, analysed its topography, hydrologic regime, water chemistry and vegetation. LiDAR Olympia, WA, USA Email: [email protected] data indicated that the peatland is elevated nearly 3 m above the surrounding land- scape. Water table variations in the plateau were strongly associated with seasonal Present address Andrea K. Borkenhagen, Advisian, Calgary, and daily precipitation events, indicating ombrotrophy. The hydraulic gradient on the Alberta, Canada. plateau is downward through most of the year, demonstrating that precipitation is percolating vertically into deeper peat layers. In the rand, the hydraulic gradients are horizontal over much of the year, indicating that the plateau is draining through the rand to the lagg. -
A Geographical Model for the Altitudinal Zonation of Mire Types in the Uplands of Western Europe: the Example of Les Monts Du Forez in Eastern France
A geographical model for the altitudinal zonation of mire types in the uplands of western Europe: the example of Les Monts du Forez in eastern France H. Cubizolle1 and G. Thebaud2 1Lyon University, EVS-ISTHME UMR 5600 CNRS, Saint-Etienne, France 2Herbarium Institute, Blaise Pascal University, Clermont-Ferrand, France _______________________________________________________________________________________ SUMMARY The geographical distribution of mires in the oceanic mountain ranges of western Europe cannot be explained without bringing together a number of physical and human factors. In Les Monts du Forez, which are granitic and metamorphic mountains covering an area of 1800 km2 and rising to an altitude of 1634 m in the east of the French Massif Central, a series of mires on long slopes reflects the effects of changing combinations of these factors with altitude. The scale of variation falls within the limits of bioclimatic levels and is manifest as: the absence of mires at foothill levels below 900 m; small mires of anthropogenic origin and remnant peat at lower mountain levels between 900 m and 1100 m; peat systems where evolution has been more or less affected by human intervention at median mountain levels between 1100 m and 1250 m; large ombrotrophic mires, often naturally convex and dating from the first half of the Holocene epoch, at upper mountain levels between 1250 m and 1450 m; and small established mires that are more or less directly linked to human intervention at sub-alpine levels above 1450 m. The role of human societies appears to dominate, with exceptions in the upper mountain levels. Human influence presents in two forms, both of which are related to the old traditional farming methods of the region: the first is destruction of mires, mostly by drainage, and the second is mire creation through modifications of the hydrology of the valley floor and the vegetation cover. -
Hydrology and Dissolved Organic Carbon Biogeochemistry in an Ombrotrophic Bog
HYDROLOGICAL PROCESSES Hydrol. Process. 15, 3151–3166 (2001) DOI: 10.1002/hyp.322 Hydrology and dissolved organic carbon biogeochemistry in an ombrotrophic bog C. J. D. Fraser, N. T. Roulet* and T. R. Moore Department of Geography and the Centre for Global and Climate Change Research, McGill University, Burnside Hall, 805 Sherbrooke St. W., Montr´eal, Qu´e., H3A 2K6 Canada Abstract: At the Mer Bleue bog, Ontario, Canada, DOC export measured at the basin outflow was 8Ð3 š 3Ð7gCm2 yr1, and DOC loading via precipitation was estimated to be 1Ð5 š 0Ð7gCm2 yr1. Discharge and DOC export calculated using a Dupuit–Forchheimer approximation compared well (within 1 g C m2 yr1) to outflow estimates of DOC export, and confirmed that outflow measurements were a suitable proxy for DOC seepage at the peatland margins. DOC export was 12% of the magnitude of the residual carbon sink measured at the peatland. The [DOC] across groundwater transects decreased with depth, and [DOC] sampled below 0Ð75 m depths remained fairly constant over the study period. However, [DOC] exported through the acrotelm (0 to 0Ð45 m peat depth) was variable, ranging from 40 mg l1 after snowmelt to 70 mg l1 during the growing season. Fluorescence analysis revealed that exported DOC was ‘allochthonous-like’, whereas DOC in the catotelm (deeper layers of peat) became more ‘autochthonous-like’ with depth. A conceptual model is developed to summarize the hydrological processes and controls which affect DOC biogeochemistry at the Mer Bleue. Copyright 2001 John Wiley & Sons, Ltd. KEY WORDS DOC; peatlands; carbon budgets; groundwater; flowpath INTRODUCTION Best estimates of global carbon (C) fluxes suggest that contemporary changes in land-use and fossil fuel emissions are sources of atmospheric C, whereas the biosphere and oceans are net sinks (Schimel, 1995). -
Historical Range of Variation Assessment for Wetland and Riparian Ecosystems, U.S
Historical Range of Variation Assessment for Wetland and Riparian Ecosystems, U.S. Forest Service Rocky Mountain Region Edward Gage and David J. Cooper United States Department of Agriculture / Forest Service Rocky Mountain Research Station General Technical Report RMRS-GTR-286WWW April 2013 Gage, Edward; Cooper, David J. 2013. Historical range of variation assessment for wetland and riparian ecosystems, U.S. Forest Service Rocky Mountain Region. Gen. Tech. Rep. RMRS-GTR-286WWW. Fort Collins, CO: U.S. Department of Agriculture, Forest Service, Rocky Mountain Research Station. 239 p. Abstract This document provides an overview of historical range of variation concepts and explores their application to wetland and riparian ecosystems in the US Forest Service Rocky Mountain Region (Region 2), which includes National Forests and National Grasslands occurring in the states of Colorado, Wyoming, Nebraska, Kansas, and South Dakota. For each of five ecosystem types—riparian areas, fens, wet meadows, salt flats, and marshes—we review key structural and functional characteristics including geomorphic setting, principal ecological drivers, classification, and dominant vegetation. In addition, we discuss anthropogenic factors known to influence the abundance or condition of each main wetland type. Keywords: wetlands, wet meadows, fens, salt flats, riparian, marshes, Colorado, Wyoming, South Dakota, Kansas, Nebraska Acknowledgments We thank the numerous individuals who provided us information, data, or critical review of this document including Barry Johnston, Bernie Bornong, Carl Chambers, Dave Bradford, Deanna Reyher, Dennis Lowry, Gay Austin, Greg Schenbeck, Jeff Redder, Jim Maxwell, Joe Neal, Joe Rocchio, Kathy Carsey, Ken Kanaan, Les Dobson, Les Gonyer, Michele Girard, Ray Zubick, Dave Ode, Gerry Steinauer, George Jones, Kelly Kindscher, Dean Erhard, Mike Johnson, Joanna Lemly, Kathy Roche, Rod Chimner, John Proctor, and Mike Menaffe. -
Poor Conifer Swamp Communitypoor Conifer Abstract Swamp, Page 1
Poor Conifer Swamp CommunityPoor Conifer Abstract Swamp, Page 1 Community Range Prevalent or likely prevalent Photo by Joshua G. Cohen Infrequent or likely infrequent Absent or likely absent Overview: Poor conifer swamp is a nutrient-poor, forested peatlands occur across their range along north-south peatland characterized by acidic, saturated peat, and and east-west climatic gradients (Glaser 1992). In the prevalence of conifer trees, Sphagnum mosses, and Michigan, poor conifer swamps are common throughout ericaceous shrubs. The community is located in depressions the northern Lower Peninsula and the Upper Peninsula in glacial outwash and sandy glacial lakeplains and in and are less common south of the climatic tension kettles on pitted outwash and depressions on moraines. zone (Amon et al. 2002). Forested peatlands are Poor conifer swamp occasionally occurs on floating mats particularly abundant in the eastern Upper Peninsula. on the margins of lakes and ponds. Fire occurs naturally Poor conifer swamps and other peatlands occur where during drought periods and creates even-aged, often excess moisture is abundant (where precipitation is monospecific, stands of Picea mariana (black spruce). greater than evapotranspiration) (Halsey and Vitt 2000, Windthrow, beaver flooding, and insect defoliation are Mitsch and Gosselink 2000). Conditions suitable for the also important disturbance factors influencing species development of peatlands have occurred in the northern composition and structure. Lake States for the past 3,000-6,000 years following climatic cooling (Boelter and Verry 1977, Heinselman Global and State Rank: G4/S4 1970, Miller and Futyma 1987). Sphagnum dominated peatlands reached their current extent 2,000-3,000 years Range: Poor conifer swamps are a frequent forested ago (Halsey and Vitt 2000).