Cardinal Design Project
Total Page:16
File Type:pdf, Size:1020Kb
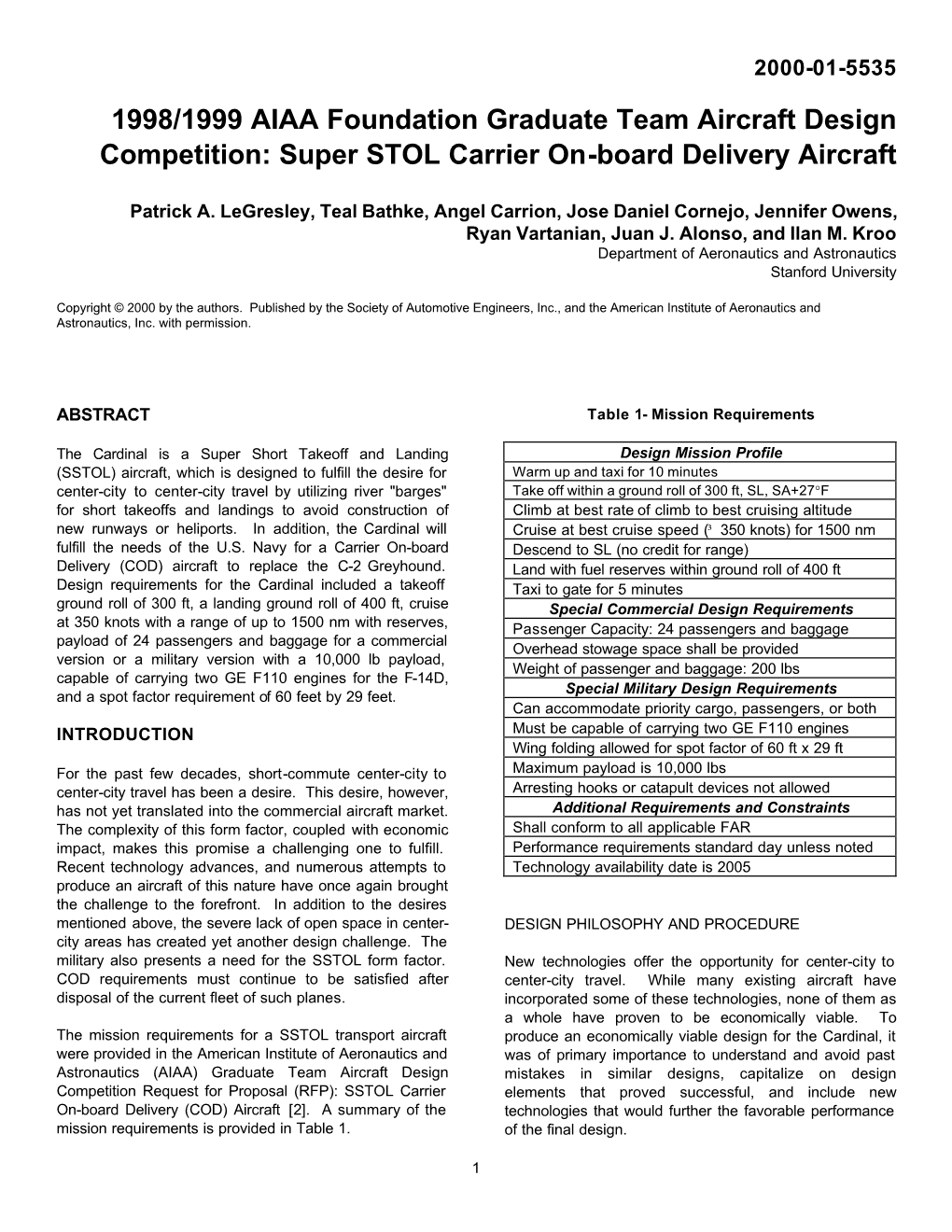
Load more
Recommended publications
-
VTOL Hybrids: Tiltrotors Are Gaining Acceptance Nihad E Daidzic,, Ph.D., Sc.D
Minnesota State University, Mankato From the SelectedWorks of Nihad E. Daidzic, Dr.-Ing., D.Sc., ATP, CFII, MEI February, 2017 VTOL hybrids: Tiltrotors are gaining acceptance Nihad E Daidzic,, Ph.D., Sc.D. This work is licensed under a Creative Commons CC_BY-NC-ND International License. Available at: https://works.bepress.com/nihad-daidzic/30/ VTOL HYBRIDS Tiltrotors are gaining acceptance Led by Bell’s XV3 and XV15 and military service-proven Bell-Boeing V22, Leonardo will market the AW609 with other wing & rotor hybrids forthcoming. ry-wing VTOL aircraft that can hov- er, ly vertically up and down, and ly forward, sideways and backward – simply amazing machines. Heli- copters produce thrust as a vector component of the total lift force by effectively tilting the main rotor disc. Gyroplanes, on the other hand, 1st lown in 1923, are hybrids between helicopters and airplanes in which case the lift-producing rotary-wing is in the constant state of autorota- tion (windmilling), while horizontal thrust is normally produced by an internal combustion engine driving conventional propellers. So why do Photo courtesy Leonardo we need yet another airplane-heli- Leonardo AW609, jointly developed by Bell Helicopter and AgustaWestland, holds promise to be copter crossbreed? We need it be- the 1st tiltrotor aircraft certified for civilian operations. Configuration options include corporate, cause helicopters and gyroplanes SAR and EMS. Projected max range is 1100 nm with aux fuel tank, cruise speed of up to 275 kts. are limited by low cruising airspeeds and ranges, while airplanes are not By Nihad Daidzic, PhD, ScD cipal innovations in the published VTOL-capable. -
PROGRAM #Aiaascitech
9–13 JANUARY 2017 GRAPEVINE, TX Addressing Full Spectrum Disruptions Across the Global Aerospace Community PROGRAM www.aiaa-SciTech.org #aiaaSciTech 17-4000 WHAT’S IMPOSSIBLE TODAY WON’T BE TOMORROW. AT LOCKHEED MARTIN, WE’RE ENGINEERING A BETTER TOMORROW. We are partnering with our customers to accelerate manufacturing innovation from the laboratory to production. We push the limits in additive manufacturing, advanced materials, digital manufacturing and next generation electronics. Whether it is solving a global crisis like the need for clean drinking water or travelling even deeper into space, advanced manufacturing is opening the doors to the next great human revolution. Learn more at lockheedmartin.com © 2014 LOCKHEED MARTIN CORPORATION VC377_164 Executive Steering Committee AIAA SciTech Forum Welcome Welcome to the 2017 AIAA Science and Technology Forum and Exposition (AIAA SciTech Forum) – the world’s largest event for aerospace research, development, and technology! Only here will you find the diversity of topics, caliber of speakers, and level of discourse about issues that directly impact your work, your career, and your industry – we are confident you will leave Grapevine prepared to shape the future of aerospace in new and exciting ways. Chuck Gustafson Jill Marlowe By bringing together 11 aerospace science and technology conferences, and by attracting attendees The Aerospace NASA Langley from across academia, industry, and government, AIAA SciTech will give you an unparalleled Corporation Research Center opportunity to hear from industry thought leaders, interact with your peers, and begin the inspired exchange of ideas that so often leads to breakthroughs in our community. Our organizing committee has worked hard over the past year to ensure that our plenary sessions examine some of the most critical issues facing aerospace today, especially the role that disruption plays in our community for better or worse. -
Future of Vertical Flight
www.vtol.org Kenneth Swartz, Regional Director – Americas The Vertical Flight Society www.vtol.org | [email protected] Kitty Hawk Cora © Vertical Flight Society: CC-BY-SA 4.0 © Vertical Flight Society: CC-BY-SA 4.0 Released March 2018 1 www.vtol.org . Founded as “The American Helicopter Society, Inc.” 75 years ago in Connecticut on Feb. 25, 1943 – “For the purpose of collecting, compiling and disseminating information concerning the helicopter” – Sikorsky Aircraft received its order for the first American helicopters on January 5, 1943 (28 XR-4 helicopters) . The first and longest-serving helicopter non-profit Sikorsky XR-4 helicopter – Founding members Igor Sikorsky, Arthur Young, Frank Piasecki, Courtesy of Sikorsky Aircraft Corp. Stanley Hiller, Reggie Brie, A.A. Griffiths, etc. – Included engineers, pilots, operators and presidents from industry, academia and government in Allied countries . Now 6,000 individual and 95 corporate members . Advancing vertical flight worldwide First Annual AHS Awards Banquet Born with the American Helicopter Industry Oct. 7, 1944 © Vertical Flight Society: CC-BY-SA 4.0 2 www.vtol.org © Vertical Flight Society: CC-BY-SA 4.0 3 www.vtol.org . The international professional society for those working to advance vertical flight – Founded in 1943 as the American Helicopter Society – Everything from VTOL MAVs/UAS to helicopters and eVTOL to STOVL (everything vertical except rockets) CFD of Joby S4, Aug 2015 . Expands knowledge about vertical flight technology and promotes its application around the world . Advances safety and acceptability . Advocates for vertical flight R&D funding . Helps educate and support today’s and tomorrow’s vertical flight engineers and leaders VFF Scholarship Winners at AHS Forum 71, May 2015 © Vertical Flight Society: CC-BY-SA 4.0 4 www.vtol.org . -
FINAL PROGRAM #Aiaascitech
4–8 JANUARY 2016 SAN DIEGO, CA The Largest Event for Aerospace Research, Development, and Technology FINAL PROGRAM www.aiaa-SciTech.org #aiaaSciTech 16-928 WHAT’S IMPOSSIBLE TODAY WON’T BE TOMORROW. AT LOCKHEED MARTIN, WE’RE ENGINEERING A BETTER TOMORROW. We are partnering with our customers to accelerate manufacturing innovation from the laboratory to production. We push the limits in additive manufacturing, advanced materials, digital manufacturing and next generation electronics. Whether it is solving a global crisis like the need for clean drinking water or travelling even deeper into space, advanced manufacturing is opening the doors to the next great human revolution. Learn more at lockheedmartin.com © 2014 LOCKHEED MARTIN CORPORATION VC377_164 Executive Steering Committee AIAA SciTech 2016 2O16 Welcome Welcome to the AIAA Science and Technology Forum and Exposition 2016 (AIAA SciTech 2016) – the world’s largest event for aerospace research, development, and technology. We are confident that you will come away from San Diego inspired and with the tools necessary to continue shaping the future of aerospace in new and exciting ways. From hearing preeminent industry thought leaders, to attending sessions where cutting- edge research will be unveiled, to interacting with peers – this will be a most fulfilling week! Our organizing committee has worked hard over the past year to ensure that our plenary sessions examine the most critical issues facing aerospace today, such as aerospace science and Richard George Lesieutre technology policy, lessons learned from a half century of aerospace innovation, resilient design, Christiansen The Pennsylvania and unmanned aerial systems. We will also focus on how AIAA and other stakeholders in State University Sierra Lobo, Inc. -
Design Optimization of High-Speed Proprotor Aircraft
f /,W-.-o5 NASA Technical Memorandum 103988 Design Optimization of High-Speed Proprotor Aircraft David R. Schleicher, James D. Phillips, and Kevin B. Carbajal (NASA-TM-I03988) DESIGN N94-26151 OPTIMIZATION OF HIGH-SPEEO PROPROTOR AIRCRAFT (NASA) 4O p Unclas G3/05 0208973 April 1993 National Aeronautics and Space Adminisb'ation "TL_',.h.._ NASA Technical Memorandum 103988 Design Optimization of High-Speed Proprotor Aircraft David R. Schleicher, James D. Phillips, and Kevin B. Carbajal Ames Research Center, Moffett Field, California April 1993 I_d/LqA National Aeronautics and Space Administration Ames Research Center Moffett Field, California 94035-1000 Contents Page Summary ................................................................................................................................................................................ 1 Nomenclature ......................................................................................................................................................................... I 1. Introduction ................................................................................................................................................................. I 2. Configurations and Mission ....................................................................................................................................... 2 3. Aircraft Synthesls ....................................................................................................................................................... -
Aviation Week & Space Technology
Airbus Sees Greener CEO Q&A What Led to USAF’s Future in Hydrogen RICH MEDIA Safran’s Petitcolin RICH MEDIA NGAD Demonstrator EXCLUSIVE EXCLUSIVE $14.95 SEPTEMBER 28-OCTOBER 11, 2020 QUIET REVOLUTION Digital Edition Copyright Notice The content contained in this digital edition (“Digital Material”), as well as its selection and arrangement, is owned by Informa. and its affiliated companies, licensors, and suppliers, and is protected by their respective copyright, trademark and other proprietary rights. Upon payment of the subscription price, if applicable, you are hereby authorized to view, download, copy, and print Digital Material solely for your own personal, non-commercial use, provided that by doing any of the foregoing, you acknowledge that (i) you do not and will not acquire any ownership rights of any kind in the Digital Material or any portion thereof, (ii) you must preserve all copyright and other proprietary notices included in any downloaded Digital Material, and (iii) you must comply in all respects with the use restrictions set forth below and in the Informa Privacy Policy and the Informa Terms of Use (the “Use Restrictions”), each of which is hereby incorporated by reference. Any use not in accordance with, and any failure to comply fully with, the Use Restrictions is expressly prohibited by law, and may result in severe civil and criminal penalties. Violators will be prosecuted to the maximum possible extent. You may not modify, publish, license, transmit (including by way of email, facsimile or other electronic means), transfer, sell, reproduce (including by copying or posting on any network computer), create derivative works from, display, store, or in any way exploit, broadcast, disseminate or distribute, in any format or media of any kind, any of the Digital Material, in whole or in part, without the express prior written consent of Informa. -
MODELING of TILTWING AIRCRAFT DYNAMICS AS LINEAR SYSTEM 1. Introduction Understanding and Accurate Modeling of Systems Behavior
MODELING OF TILTWING AIRCRAFT DYNAMICS AS LINEAR SYSTEM JOHANNA HOLSTEN* PHILIPP HARTMANN* DIETER MOORMANN* *Institute of Flight System Dynamics, RWTH Aachen University, Wüllnerstr. 7, 52064 Aachen, Germany [email protected] Abstract In this paper the nonlinear system dynamics of a tiltwing aircraft is linearized at a variation of operating points. Effect of linearization on dynamic behavior is analyzed in comparison to the original nonlinear model. Aircraft in tiltwing configuration combine the advantages of helicopters, such as hovering and vertical take-off and landing capabilities, with the advantages of conventional fixed- wing aircraft. During transition of tiltwing aircraft between hovering and aerodynamic horizontal forward flight, tilting the wing from vertical to horizontal position (and vice versa) poses a significant change in configuration. In combination with the given large velocity range this influences the control device effectiveness significantly. At the same time, tilting the wing provides an additional control variable. These aspects make designing a tiltwing flight controller complex. For controller design and analysis, linearization of the system dynamics as LTI model allows direct analysis of its stability margins. In this paper we show that the linear model of the tiltwing aircraft represents the nonlinear system sufficiently for controller analysis and design. Additionally it provides insight into changing dynamics and allows further analysis in the frequency domain. Keywords Tiltwing Aircraft; Flight System Dynamics. 1. Introduction Understanding and accurate modeling of systems behavior is a necessity when aiming at robust control performance. A linear model in contrast to a nonlinear model allows direct analysis and simplifies the control design process. Furthermore, state-space representations are more suitable for numerical calculations. -
The a Graduate Team Aircraft Design Stanford University
The A Graduate Team Aircraft Design June 1, 1999 Stanford University The Cardinal: A 1999 AIAA Graduate Team Aircraft Design TH E St a n f o r d Un iv er s it y A 1999 AIAA GRADUATE TEAM AIRCRAFT PROPOSAL PROJECT LEADER: Patrick LeGresley Jose Daniel Cornejo AIAA Member Number 144155 AIAA Member Number 143144 MS Expected June 1999 MS Expected March 2000 [email protected] [email protected] Teal Bathke Jennifer Owens AIAA Member Number 183891 AIAA Member Number 113704 MS Expected June 2000 MS Expected December 1999 [email protected] [email protected] Angel Carrion Ryan Vartanian AIAA Member Number 183513 AIAA Member Number 154990 MS Expected March 2000 MS Expected June 1999 [email protected] [email protected] ADVISORS: Dr. Juan Alonso Dr. Ilan Kroo Assistant Professor Professor Stanford University Aeronautics & Astronautics Stanford University Aeronautics & Astronautics [email protected] [email protected] “When I was a student in college, just flying an airplane seemed a dream…” ~Charles Lindbergh JUNE 1, 1999 “When I was a student in college, just flying an airplane seemed a dream…” June 1, 1999 Signature Page ~Charles Lindbergh The Cardinal: A 1999 AIAA Graduate Team Aircraft Design EXECUTIVE SUMMARY Commuting from center-city to center-city is not viable with existing aircraft due to the lack of available runways in metropolitan areas for airplanes and higher expense of helicopter service. The Cardinal is a Super Short Takeoff and Landing (SSTOL) aircraft which attempts to fill this gap. The major goal of this airplane design is to fulfill the desire for center-city to center-city travel by utilizing river “barges” for short takeoffs and landings to avoid construction of new runways or heliports. -
What Is a Tiltrotor? a Fundamental Reexamination of the Tiltrotor Aircraft Design Space
What is a Tiltrotor? A Fundamental Reexamination of the Tiltrotor Aircraft Design Space Larry A. Young NASA Ames Research Center, Moffett Field, CA 94035 Abstract The objective of this work is to fundamentally reexamine the design space of tiltrotor aircraft beyond the very successful conventional vehicle configuration with tractor-type twin proprotors that are nacelle- mounted at the wing tips. This work seeks to define a broad aircraft design space for alternate tiltrotor configurations. It is hoped that this work will not only provide design inspiration for future aircraft developers, but also help realize in the future new applications and missions for both passenger-carrying vehicles and vertical lift UAVs. Introduction After a multi-decade development effort, the first production tiltrotor aircraft, the MV/CV-22 Osprey, has become a key element of U.S. military aviation. After its own long fitful development effort, the AW-609, the first civil tiltrotor aircraft, seems to be finally nearing certification. Design studies continue to show the promise of large civil tiltrotor aircraft for commercial passenger transport – particularly as such aircraft might help enable significant National Airspace System (NAS) throughput increases and delay reduction through their VTOL/runway-independent nature. Tiltrotor aircraft have finally arrived. Meanwhile, though, a recent resurgence of development activity in compound helicopters, notably the X2 and X3 compound helicopters, has narrowed the speed/range advantage of tiltrotor aircraft over such vehicles. In parallel with these advances in manned rotary-wing aircraft, aviation as a whole is poised to see a rapid expansion in uninhabited VTOL UAV’s supporting new civilian/commercial missions/applications. -
Vertical Lift – Not Just for Terrestrial Flight
Vertical Lift – Not Just For Terrestrial Flight Larry A. Young Army/NASA Rotorcraft Division Ames Research Center Moffett Field, CA Abstract Autonomous vertical lift vehicles hold considerable potential for supporting planetary science and exploration missions. This paper discusses several technical aspects of vertical lift planetary aerial vehicles in general, and specifically addresses technical challenges and work to date examining notional vertical lift vehicles for Mars, Titan, and Venus exploration. for planetary exploration and science Introduction missions (fig. 1). Initial results have been quite promising. As a result of this The next few years promise a unique early work, the utility of rotorcraft, convergence of NASA aeronautics and VTOL vehicles, and hybrid airships for space programs. NASA planetary Mars exploration and planetary science science missions are becoming missions as a whole can be technically increasingly more sophisticated. justified as follows. Manned and robotic exploration of the solar system planets would be greatly Why vertical lift vehicles for planetary enhanced through the development and exploration? For the same reasons why use of robotic aerial vehicles. Since the these vehicles are such flexible aerial 1970’s a number of Mars (fixed-wing) platforms for terrestrial exploration and Airplane concepts have been proposed transportation: the ability to hover and for Mars exploration. fly at low-speeds and to take-off and land at unprepared remote sites. Further, The Army/NASA Rotorcraft Division -- autonomous vertical lift planetary aerial in collaboration with the Center for Mars vehicles (PAVs) would have the Exploration -- at NASA Ames has been following specific advantages and performing initial conceptual design capabilities for planetary exploration: studies of Martian autonomous rotorcraft • Hover and low-speed flight AHS/AIAA/RaeS/SAE International Powered capability would enable detailed Lift Conference, Arlington, VA, October 30- November 1, 2000. -
Tiltotor Thrust Control
THRUST CONTROL OF VTOL AIRCRAFT – PART DEUX DANIEL C. DUGAN [email protected] Aero Engineer/Research Pilot´‡ NASA Ames Research Center Moffett Field, California ABSTRACT Thrust control of Vertical Takeoff and Landing (VTOL) aircraft has always been a debatable issue. In most cases, it comes down to the fundamental question of throttle versus collective. Some aircraft used throttle(s), with a fore and aft longitudinal motion, some had collectives, some have used Thrust Levers where the protocol is still “Up is Up and Down is Down,” and some have incorporated both throttles and collectives when designers did not want to deal with the Human Factors issues. There have even been combinations of throttles that incorporated an arc that have been met with varying degrees of success. A previous review was made of nineteen designs without attempting to judge the merits of the controller. Included in this paper are twelve designs entered in competition for the 1961 Tri-Service VTOL transport. Entries were from a Bell/Lockheed tiltduct, a North American tiltwing, a Vanguard liftfan, and even a Sikorsky tiltwing. Additional designs were submitted from Boeing Wichita (direct lift), Ling-Temco-Vought with its XC-142 tiltwing, Boeing Vertol’s tiltwing, Mcdonnell’s compound and tiltwing, and the Douglas turboduct and turboprop designs. A private party submitted a re-design of the Breguet 941 as a VTOL transport. It is important to document these 53 year-old designs to preserve a part of this country’s aviation heritage. INTRODUCTION During the design phase of an aircraft, the control of Vertical Takeoff and Landing (VTOL) aircraft, thrust should be a straightforward process. -
Design and Control of an Experimental Tiltwing Aircraft
NASA/CR—2017–219456 Design and Control of an Experimental Tiltwing Aircraft Linnea Persson KTH Royal Institute of Technology Ames Research Center, Moffett Field, California Ben Lawrence San Jose State University Research Foundation Ames Research Center, Moffett Field, California Click here: Press F1 key (Windows) or Help key (Mac) for help March 2017 This page is required and contains approved text that cannot be changed. NASA STI Program ... in Profile Since its founding, NASA has been dedicated • CONFERENCE PUBLICATION. to the advancement of aeronautics and space Collected papers from scientific and science. The NASA scientific and technical technical conferences, symposia, seminars, information (STI) program plays a key part in or other meetings sponsored or co- helping NASA maintain this important role. sponsored by NASA. The NASA STI program operates under the • SPECIAL PUBLICATION. Scientific, auspices of the Agency Chief Information technical, or historical information from Officer. It collects, organizes, provides for NASA programs, projects, and missions, archiving, and disseminates NASA’s STI. The often concerned with subjects having NASA STI program provides access to the NTRS substantial public interest. Registered and its public interface, the NASA Technical Reports Server, thus providing one of • TECHNICAL TRANSLATION. the largest collections of aeronautical and space English-language translations of foreign science STI in the world. Results are published in scientific and technical material pertinent to both non-NASA channels and by NASA in the NASA’s mission. NASA STI Report Series, which includes the following report types: Specialized services also include organizing and publishing research results, distributing • TECHNICAL PUBLICATION. Reports of specialized research announcements and feeds, completed research or a major significant providing information desk and personal search phase of research that present the results of support, and enabling data exchange services.