A Molecular Characterization of Agonists That Bind to Hco-UNC-49, a GABA
Total Page:16
File Type:pdf, Size:1020Kb
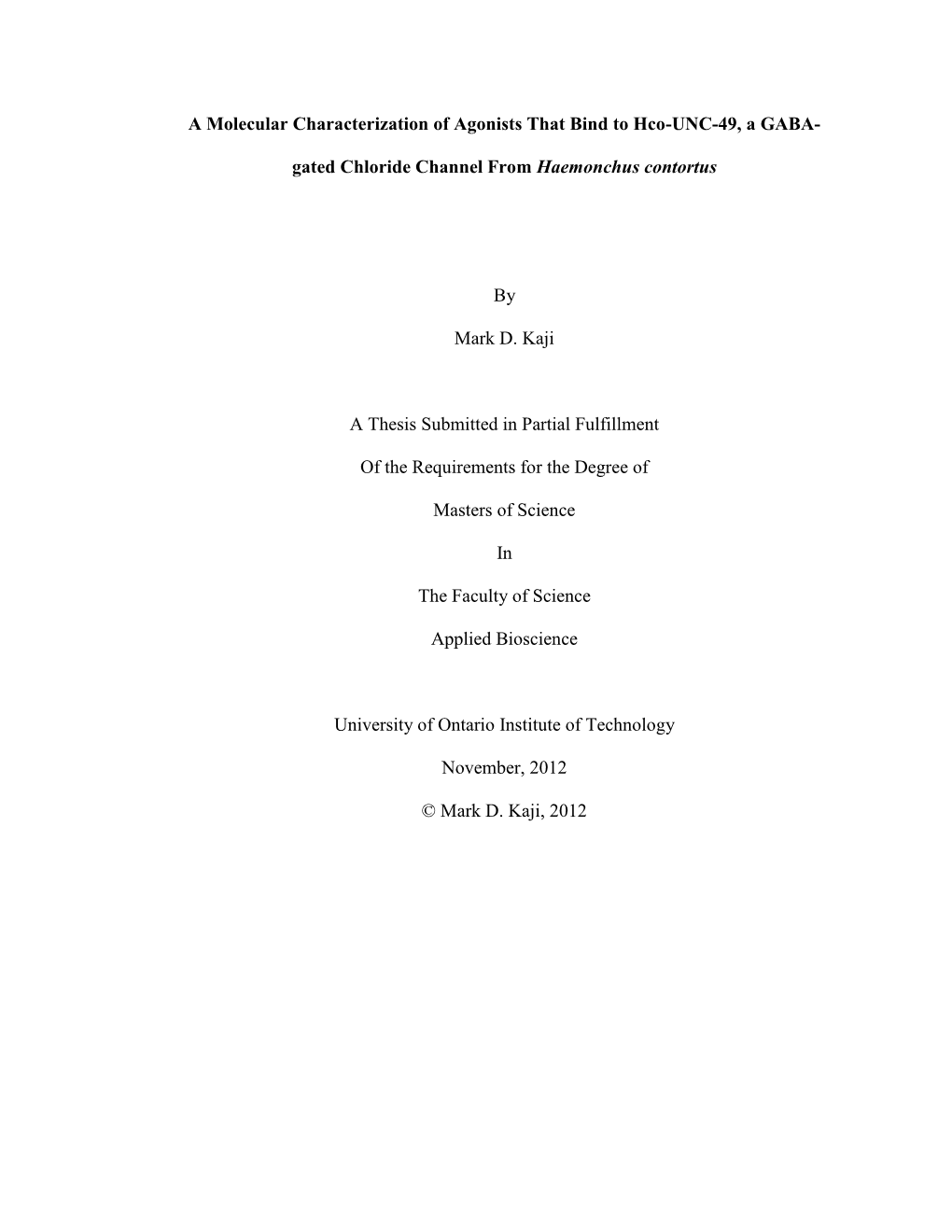
Load more
Recommended publications
-
Aldrich FT-IR Collection Edition I Library
Aldrich FT-IR Collection Edition I Library Library Listing – 10,505 spectra This library is the original FT-IR spectral collection from Aldrich. It includes a wide variety of pure chemical compounds found in the Aldrich Handbook of Fine Chemicals. The Aldrich Collection of FT-IR Spectra Edition I library contains spectra of 10,505 pure compounds and is a subset of the Aldrich Collection of FT-IR Spectra Edition II library. All spectra were acquired by Sigma-Aldrich Co. and were processed by Thermo Fisher Scientific. Eight smaller Aldrich Material Specific Sub-Libraries are also available. Aldrich FT-IR Collection Edition I Index Compound Name Index Compound Name 3515 ((1R)-(ENDO,ANTI))-(+)-3- 928 (+)-LIMONENE OXIDE, 97%, BROMOCAMPHOR-8- SULFONIC MIXTURE OF CIS AND TRANS ACID, AMMONIUM SALT 209 (+)-LONGIFOLENE, 98+% 1708 ((1R)-ENDO)-(+)-3- 2283 (+)-MURAMIC ACID HYDRATE, BROMOCAMPHOR, 98% 98% 3516 ((1S)-(ENDO,ANTI))-(-)-3- 2966 (+)-N,N'- BROMOCAMPHOR-8- SULFONIC DIALLYLTARTARDIAMIDE, 99+% ACID, AMMONIUM SALT 2976 (+)-N-ACETYLMURAMIC ACID, 644 ((1S)-ENDO)-(-)-BORNEOL, 99% 97% 9587 (+)-11ALPHA-HYDROXY-17ALPHA- 965 (+)-NOE-LACTOL DIMER, 99+% METHYLTESTOSTERONE 5127 (+)-P-BROMOTETRAMISOLE 9590 (+)-11ALPHA- OXALATE, 99% HYDROXYPROGESTERONE, 95% 661 (+)-P-MENTH-1-EN-9-OL, 97%, 9588 (+)-17-METHYLTESTOSTERONE, MIXTURE OF ISOMERS 99% 730 (+)-PERSEITOL 8681 (+)-2'-DEOXYURIDINE, 99+% 7913 (+)-PILOCARPINE 7591 (+)-2,3-O-ISOPROPYLIDENE-2,3- HYDROCHLORIDE, 99% DIHYDROXY- 1,4- 5844 (+)-RUTIN HYDRATE, 95% BIS(DIPHENYLPHOSPHINO)BUT 9571 (+)-STIGMASTANOL -
(12) Patent Application Publication (10) Pub. No.: US 2009/0156618 A1 Tollefson (43) Pub
US 20090156618A1 (19) United States (12) Patent Application Publication (10) Pub. No.: US 2009/0156618 A1 Tollefson (43) Pub. Date: Jun. 18, 2009 (54) 1-(1-(2-ETHOXYETHYL)-3-ETHYL-7- Related U.S. Application Data (4-METHYLPYRIDIN-2-YLAMINO) - 1H-PYRAZOLO 4.3-D PYRIMIDIN-5-YL) (60) Provisional application No. 60/735,320, filed on Nov. PPERDINE-4-CARBOXYLIC ACID AND 10, 2005. SALTS THEREOF Publication Classification (51) Int. Cl. (75) Inventor: Michael B. Tollefson, Dardenne A 6LX 3/59 (2006.01) Prairie, MO (US) C07D 487/04 (2006.01) A6IP 9/12 (2006.01) A6IP 25/00 (2006.01) Correspondence Address: A6IP 9/00 (2006.01) PFZER INC. PATENT DEPARTMENT, Bld 114 M/S 114, (52) U.S. Cl. ...................................... 514/262.1; 54.4/262 EASTERN PONT ROAD (57) ABSTRACT GROTON, CT 06340 (US) The present invention comprises 1-(1-(2-ethoxyethyl)-3- ethyl-7-(4-methylpyridin-2-ylamino)-1H-pyrazolo 4.3-d (73) Assignee: Pfizer Inc pyrimidin-5-yl)piperidine-4-carboxylic acid and its salts. The invention further comprises pharmaceutical composi tions, methods of treatment, and synthetic methods relating to (21) Appl. No.: 111558,306 1-(1-(2-ethoxyethyl)-3-ethyl-7-(4-methylpyridin-2- ylamino)-1H-pyrazolo 4.3-dipyrimidin-5-yl)piperidine-4- (22) Filed: Nov. 9, 2006 carboxylic acid and its salts. Patent Application Publication Jun. 18, 2009 Sheet 2 of 2 US 2009/0156618 A1 "SOIHZ US 2009/0156618 A1 Jun. 18, 2009 1-(1-(2-ETHOXYETHYL)-3-ETHYL-7- 1H-pyrazolo 4,3-dipyrimidin-5-yl)piperidine-4-carboxylic (4-METHYLPYRIDIN-2-YLAMINO) - acid and its pharmaceutically acceptable salts. -
ANNNNNNNNNNNNNNNNNNNN 100A 006 Left Eye Input Right Eye Input
US 20190175049A1 ( 19) United States (12 ) Patent Application Publication (10 ) Pub. No. : US 2019 /0175049 A1 Welling ( 43 ) Pub . Date : Jun . 13 , 2019 ( 54 ) TECHNIQUES FOR ANALYZING (52 ) U . S . CI. NON -VERBAL MARKERS OF CONDITIONS CPC . .. A61B 5 /04842 (2013 . 01 ) ; A61B 5 / 7289 USING ELECTROPHYSIOLOGICAL DATA (2013 . 01) ; A61B 5 /0478 ( 2013 .01 ) ; A61B 5 /7225 ( 2013. 01 ) ; G06N 20 / 10 (2019 .01 ) (71 ) Applicant: Massachusetts Institute of Technology , Cambridge , MA (US ) ( 57 ) ABSTRACT (72 ) Inventor : Caroline Welling, Hanover, NH (US ) Embodiments related to analyzing brain activity of a subject to identify signs associated with binocular rivalry . Sensed ( 21 ) Appl. No. : 16 / 206, 639 electrical activity of a subject' s brain is received over a time period while the subject is exposed to a visual stimulus. The ( 22 ) Filed : Nov. 30 , 2018 sensed electrical activity comprises a first frequency band Related U . S . Application Data associated with a first frequency of a first image presented to the subject ' s left eye , a second frequency band associated (60 ) Provisional application No .62 / 593 , 535, filed on Dec . with a second frequency of a second image presented to the 1 , 2017 subject ' s right eye . A set of events in the time period is determined based on the frequency bands, wherein an event Publication Classification is associated with a change from a previous perceptual event (51 ) Int. Ci. to a new perceptual event. A metric for the subject is A61B 5 /0484 ( 2006 .01 ) determined based on the set of events . The metric is ana A61B 5 /00 ( 2006 .01 ) lyzed to determine whether the subject exhibits signs asso GO6N 20 / 10 (2006 .01 ) ciated with a condition that is associated with binocular A61B 5 /0478 ( 2006 .01 ) rivalry . -
Campro Catalog Stable Isotope
Introduction & Welcome Dear Valued Customer, We are pleased to present to you our Stable Isotopes Catalog which contains more than three thousand (3000) high quality labeled compounds. You will find new additions that are beneficial for your research. Campro Scientific is proud to work together with Isotec, Inc. for the distribution and marketing of their stable isotopes. We have been working with Isotec for more than twenty years and know that their products meet the highest standard. Campro Scientific was founded in 1981 and we provide services to some of the most prestigious universities, research institutes and laboratories throughout Europe. We are a research-oriented company specialized in supporting the requirements of the scientific community. We are the exclusive distributor of some of the world’s leading producers of research chemicals, radioisotopes, stable isotopes and environmental standards. We understand the requirements of our customers, and work every day to fulfill them. In working with us you are guaranteed to receive: - Excellent customer service - High quality products - Dependable service - Efficient distribution The highly educated staff at Campro’s headquarters and sales office is ready to assist you with your questions and product requirements. Feel free to call us at any time. Sincerely, Dr. Ahmad Rajabi General Manager 180/280 = unlabeled 185/285 = 15N labeled 181/281 = double labeled (13C+15N, 13C+D, 15N+18O etc.) 186/286 = 12C labeled 182/282 = d labeled 187/287 = 17O labeled 183/283 = 13C labeleld 188/288 = 18O labeled 184/284 = 16O labeled, 14N labeled 189/289 = Noble Gases Table of Contents Ordering Information.................................................................................................. page 4 - 5 Packaging Information .............................................................................................. -
(12) Patent Application Publication (10) Pub. No.: US 2007/0254920 A1 Delong Et Al
US 20070254920A1 (19) United States (12) Patent Application Publication (10) Pub. No.: US 2007/0254920 A1 deLong et al. (43) Pub. Date: Nov. 1, 2007 (54) PRODRUG DERIVATIVES OF ACIDS USING (52) U.S. Cl. ......................... 514/319; 514/323: 514/573; ALCOHOLS WITH HOMOTOPIC HYDROXY 514/415: 514/443; 514/469; GROUPS AND METHODS FOR THEIR 546/196; 546/201: 546/206; PREPARATION AND USE 548/495; 549/49; 549/462 (75) Inventors: Mitchell A. deLong, Raleigh, NC (US); (57) ABSTRACT Jill M. McFadden, Chapel Hill, NC This invention relates to novel homotopic prodrugs and (US); Susan M. Royalty, Cary, NC medicaments and methods for their preparation, testing and (US); Eric J. Toone, Durham, NC use. In one embodiment, the homotopic prodrug has the (US); Jeffrey D. Yingling, Apex, NC general formula (US) Correspondence Address: O MICHAEL BEST & FRIEDRCH LLP 100 E WISCONSNAVENUE Rb, Suite 3300 --- MILWAUKEE, WI 53202 (US) wherein (73) Assignee: Aerie Pharmaceuticals, Inc., Research Triangle Park, NC (21) Appl. No.: 11/412,207 (22) Filed: Apr. 26, 2006 -- Publication Classification is a biologically-active moiety comprising a carboxylic acid functional group, and R is a homotopically-symmetrical (51) Int. Cl. alcohol bonded to the biologically-active moiety through the A6 IK 3/558 (2006.01) carboxylic acid functional group to form an ester linkage, as CO7D 409/02 (2006.01) well as optical isomers, enantiomers, pharmaceutically CO7D 405/02 (2006.01) acceptable salts, biohydrolyzable amides, esters, and imides CO7D 403/02 (2006.01) thereof and combinations thereof. US 2007/0254920 A1 Nov. 1, 2007 PRODRUG DERVATIVES OF ACDS USING WO 99/12896, and WO 99/12898.) However, such modifi ALCOHOLS WITH HOMOTOPIC HYDROXY cations have either resulted in only modest increases in GROUPS AND METHODS FOR THEIR half-life or resulted in compounds with diminished potency. -
GABAA Transmission Is a Critical Step in the Process of Triggering Homeostatic Increases in Quantal Amplitude
GABAA transmission is a critical step in the process of triggering homeostatic increases in quantal amplitude Jennifer C. Wilhelm and Peter Wenner* Department of Physiology, Emory University School of Medicine, Atlanta, GA 30322 Communicated by Lynn T. Landmesser, Case Western Reserve University, Cleveland, OH, June 23, 2008 (received for review March 1, 2008) When activity levels are altered over days, a network of cells is models suggests that reducing network activity produces a corre- capable of recognizing this perturbation and triggering several dis- sponding reduction in cellular spiking activity, thereby reducing tinct compensatory changes that should help to recover and maintain intracellular calcium levels in a postsynaptic cell. In this model, the original activity levels homeostatically. One feature commonly which we will refer to as the cell activity model, the postsynaptic cell observed after activity blockade has been a compensatory increase in senses changes in intracellular calcium levels as a measure of altered excitatory quantal amplitude. The sensing machinery that detects activity and triggers compensatory changes in mPSC amplitude. altered activity levels is a central focus of the field currently, but thus However, the studies that have inspired this model not only block far it has been elusive. The vast majority of studies that reduce spiking activity, they also block or reduce neurotransmitter binding network activity also reduce neurotransmission. We address the to its receptor and any associated downstream signaling cascades. possibility that reduced neurotransmission can trigger increases in Thus, it remains possible that neurotransmission is a critical step in quantal amplitude. In this work, we blocked glutamatergic or GABAA the sensing process that triggers changes in quantal amplitude. -
Neurotransmitters-Drugs Andbrain Function.Pdf
Neurotransmitters, Drugs and Brain Function. Edited by Roy Webster Copyright & 2001 John Wiley & Sons Ltd ISBN: Hardback 0-471-97819-1 Paperback 0-471-98586-4 Electronic 0-470-84657-7 Neurotransmitters, Drugs and Brain Function Neurotransmitters, Drugs and Brain Function. Edited by Roy Webster Copyright & 2001 John Wiley & Sons Ltd ISBN: Hardback 0-471-97819-1 Paperback 0-471-98586-4 Electronic 0-470-84657-7 Neurotransmitters, Drugs and Brain Function Edited by R. A. Webster Department of Pharmacology, University College London, UK JOHN WILEY & SONS, LTD Chichester Á New York Á Weinheim Á Brisbane Á Singapore Á Toronto Neurotransmitters, Drugs and Brain Function. Edited by Roy Webster Copyright & 2001 John Wiley & Sons Ltd ISBN: Hardback 0-471-97819-1 Paperback 0-471-98586-4 Electronic 0-470-84657-7 Copyright # 2001 by John Wiley & Sons Ltd. Bans Lane, Chichester, West Sussex PO19 1UD, UK National 01243 779777 International ++44) 1243 779777 e-mail +for orders and customer service enquiries): [email protected] Visit our Home Page on: http://www.wiley.co.uk or http://www.wiley.com All Rights Reserved. No part of this publication may be reproduced, stored in a retrieval system, or transmitted, in any form or by any means, electronic, mechanical, photocopying, recording, scanning or otherwise, except under the terms of the Copyright, Designs and Patents Act 1988 or under the terms of a licence issued by the Copyright Licensing Agency Ltd, 90 Tottenham Court Road, London W1P0LP,UK, without the permission in writing of the publisher. Other Wiley Editorial Oces John Wiley & Sons, Inc., 605 Third Avenue, New York, NY 10158-0012, USA WILEY-VCH Verlag GmbH, Pappelallee 3, D-69469 Weinheim, Germany John Wiley & Sons Australia, Ltd. -
GABA Receptors
D Reviews • BIOTREND Reviews • BIOTREND Reviews • BIOTREND Reviews • BIOTREND Reviews Review No.7 / 1-2011 GABA receptors Wolfgang Froestl , CNS & Chemistry Expert, AC Immune SA, PSE Building B - EPFL, CH-1015 Lausanne, Phone: +41 21 693 91 43, FAX: +41 21 693 91 20, E-mail: [email protected] GABA Activation of the GABA A receptor leads to an influx of chloride GABA ( -aminobutyric acid; Figure 1) is the most important and ions and to a hyperpolarization of the membrane. 16 subunits with γ most abundant inhibitory neurotransmitter in the mammalian molecular weights between 50 and 65 kD have been identified brain 1,2 , where it was first discovered in 1950 3-5 . It is a small achiral so far, 6 subunits, 3 subunits, 3 subunits, and the , , α β γ δ ε θ molecule with molecular weight of 103 g/mol and high water solu - and subunits 8,9 . π bility. At 25°C one gram of water can dissolve 1.3 grams of GABA. 2 Such a hydrophilic molecule (log P = -2.13, PSA = 63.3 Å ) cannot In the meantime all GABA A receptor binding sites have been eluci - cross the blood brain barrier. It is produced in the brain by decarb- dated in great detail. The GABA site is located at the interface oxylation of L-glutamic acid by the enzyme glutamic acid decarb- between and subunits. Benzodiazepines interact with subunit α β oxylase (GAD, EC 4.1.1.15). It is a neutral amino acid with pK = combinations ( ) ( ) , which is the most abundant combi - 1 α1 2 β2 2 γ2 4.23 and pK = 10.43. -
Thesis Rests with Its Author
University of Bath PHD A modelling study of the ligand-gated ion-channel superfamily of receptors Cockcroft, Victor Barrie Award date: 1992 Awarding institution: University of Bath Link to publication Alternative formats If you require this document in an alternative format, please contact: [email protected] General rights Copyright and moral rights for the publications made accessible in the public portal are retained by the authors and/or other copyright owners and it is a condition of accessing publications that users recognise and abide by the legal requirements associated with these rights. • Users may download and print one copy of any publication from the public portal for the purpose of private study or research. • You may not further distribute the material or use it for any profit-making activity or commercial gain • You may freely distribute the URL identifying the publication in the public portal ? Take down policy If you believe that this document breaches copyright please contact us providing details, and we will remove access to the work immediately and investigate your claim. Download date: 08. Oct. 2021 A MODELLING STUDY OF THE LIGAND-GATED ION-CHANNEL SUPERFAMILY OF RECEPTORS. submitted by Victor Barrie Cockcroft for the degree of Ph.D. at the University of Bath 1992 Copyright Attention is drawn to the fact that the copyright of this thesis rests with its author. This copy of the thesis has been supplied on the condition that anyone who con sults it is understood to recognize that its copyright rests with its author and that no quotation from the thesis and no information derived from it may be published with out the prior written consent of the author. -
Cell Surface Mobility of GABAB Receptors Saad Bin
Cell surface mobility of GABAB receptors Saad Bin Hannan September 2011 A thesis submitted in fulfilment of the requirements for the degree of Doctor of Philosophy of the University College London Department of Neuroscience, Physiology, and Pharmacology University College London Gower Street London WC1E 6BT UK Declaration ii ‘I, Saad Hannan confirm that the work presented in this thesis is my own. Where information has been derived from other sources, I confirm that this has been indicated in the thesis.' ____________________ Saad Hannan September 2011 To Ammu, Abbu, Polu Abstract ivi Abstract Type-B γ-aminobutyric acid receptors (GABABRs) are important for mediating slow inhibition in the central nervous system and the kinetics of their internalisation and lateral mobility will be a major determinant of their signalling efficacy. Functional GABABRs require R1 and R2 subunit co-assembly, but how heterodimerisation affects the trafficking kinetics of GABABRs is unknown. Here, an α- bungarotoxin binding site (BBS) was inserted into the N-terminus of R2 to monitor receptor mobility in live cells. GABABRs are internalised via clathrin- and dynamin- dependent pathways and recruited to endosomes. By mutating the BBS, a new technique was developed to differentially track R1a and R2 simultaneously, revealing the subunits internalise as heteromers and that R2 dominantly-affects constitutive internalisation of GABABRs. Notably, the internalisation profile of R1aR2 heteromers, but not R1a homomers devoid of their ER retention motif (R1ASA), is similar to R2 homomers in heterologous systems. The internalisation of R1aASA was slowed to that of R2 by mutating a di-leucine motif in the R1 C-terminus, indicating a new role for heterodimerisation, whereby R2 subunits slow the internalization of surface GABABRs. -
Isoguvacine Hydrochloride | Medchemexpress
Inhibitors Product Data Sheet Isoguvacine hydrochloride • Agonists Cat. No.: HY-100810 CAS No.: 68547-97-7 Molecular Formula: C₆H₁₀ClNO₂ • Molecular Weight: 163.6 Screening Libraries Target: GABA Receptor Pathway: Membrane Transporter/Ion Channel; Neuronal Signaling Storage: Powder -20°C 3 years 4°C 2 years In solvent -80°C 6 months -20°C 1 month SOLVENT & SOLUBILITY In Vitro H2O : ≥ 100 mg/mL (611.25 mM) DMSO : 25 mg/mL (152.81 mM; Need ultrasonic) * "≥" means soluble, but saturation unknown. Mass Solvent 1 mg 5 mg 10 mg Concentration Preparing 1 mM 6.1125 mL 30.5623 mL 61.1247 mL Stock Solutions 5 mM 1.2225 mL 6.1125 mL 12.2249 mL 10 mM 0.6112 mL 3.0562 mL 6.1125 mL Please refer to the solubility information to select the appropriate solvent. In Vivo 1. Add each solvent one by one: 10% DMSO >> 40% PEG300 >> 5% Tween-80 >> 45% saline Solubility: ≥ 2.08 mg/mL (12.71 mM); Clear solution 2. Add each solvent one by one: 10% DMSO >> 90% (20% SBE-β-CD in saline) Solubility: ≥ 2.08 mg/mL (12.71 mM); Clear solution 3. Add each solvent one by one: 10% DMSO >> 90% corn oil Solubility: ≥ 2.08 mg/mL (12.71 mM); Clear solution BIOLOGICAL ACTIVITY Description Isoguvacine hydrochloride is a GABA receptor agonist. IC₅₀ & Target GABA[1] In Vitro Isoguvacine binds to a mouse forebrain synaptic membrane preparation. The specific binding is displaceable by GABA, Page 1 of 2 www.MedChemExpress.com muscimol and bicuculline but not by picrotoxin or diaminobutyric acid. -
Gabaergic Signaling Linked to Autophagy Enhances Host Protection Against Intracellular Bacterial Infections
ARTICLE DOI: 10.1038/s41467-018-06487-5 OPEN GABAergic signaling linked to autophagy enhances host protection against intracellular bacterial infections Jin Kyung Kim1,2,3, Yi Sak Kim1,2,3, Hye-Mi Lee1,3, Hyo Sun Jin4, Chiranjivi Neupane 2,5, Sup Kim1,2,3, Sang-Hee Lee6, Jung-Joon Min7, Miwa Sasai8, Jae-Ho Jeong 9,10, Seong-Kyu Choe11, Jin-Man Kim12, Masahiro Yamamoto8, Hyon E. Choy 9,10, Jin Bong Park 2,5 & Eun-Kyeong Jo1,2,3 1234567890():,; Gamma-aminobutyric acid (GABA) is the principal inhibitory neurotransmitter in the brain; however, the roles of GABA in antimicrobial host defenses are largely unknown. Here we demonstrate that GABAergic activation enhances antimicrobial responses against intracel- lular bacterial infection. Intracellular bacterial infection decreases GABA levels in vitro in macrophages and in vivo in sera. Treatment of macrophages with GABA or GABAergic drugs promotes autophagy activation, enhances phagosomal maturation and antimicrobial responses against mycobacterial infection. In macrophages, the GABAergic defense is mediated via macrophage type A GABA receptor (GABAAR), intracellular calcium release, and the GABA type A receptor-associated protein-like 1 (GABARAPL1; an Atg8 homolog). Finally, GABAergic inhibition increases bacterial loads in mice and zebrafish in vivo, sug- gesting that the GABAergic defense plays an essential function in metazoan host defenses. Our study identified a previously unappreciated role for GABAergic signaling in linking antibacterial autophagy to enhance host innate defense against intracellular bacterial infection. 1 Department of Microbiology, Chungnam National University School of Medicine, Daejeon 35015, Korea. 2 Department of Medical Science, Chungnam National University School of Medicine, Daejeon 35015, Korea.