Download 2.16 MB
Total Page:16
File Type:pdf, Size:1020Kb
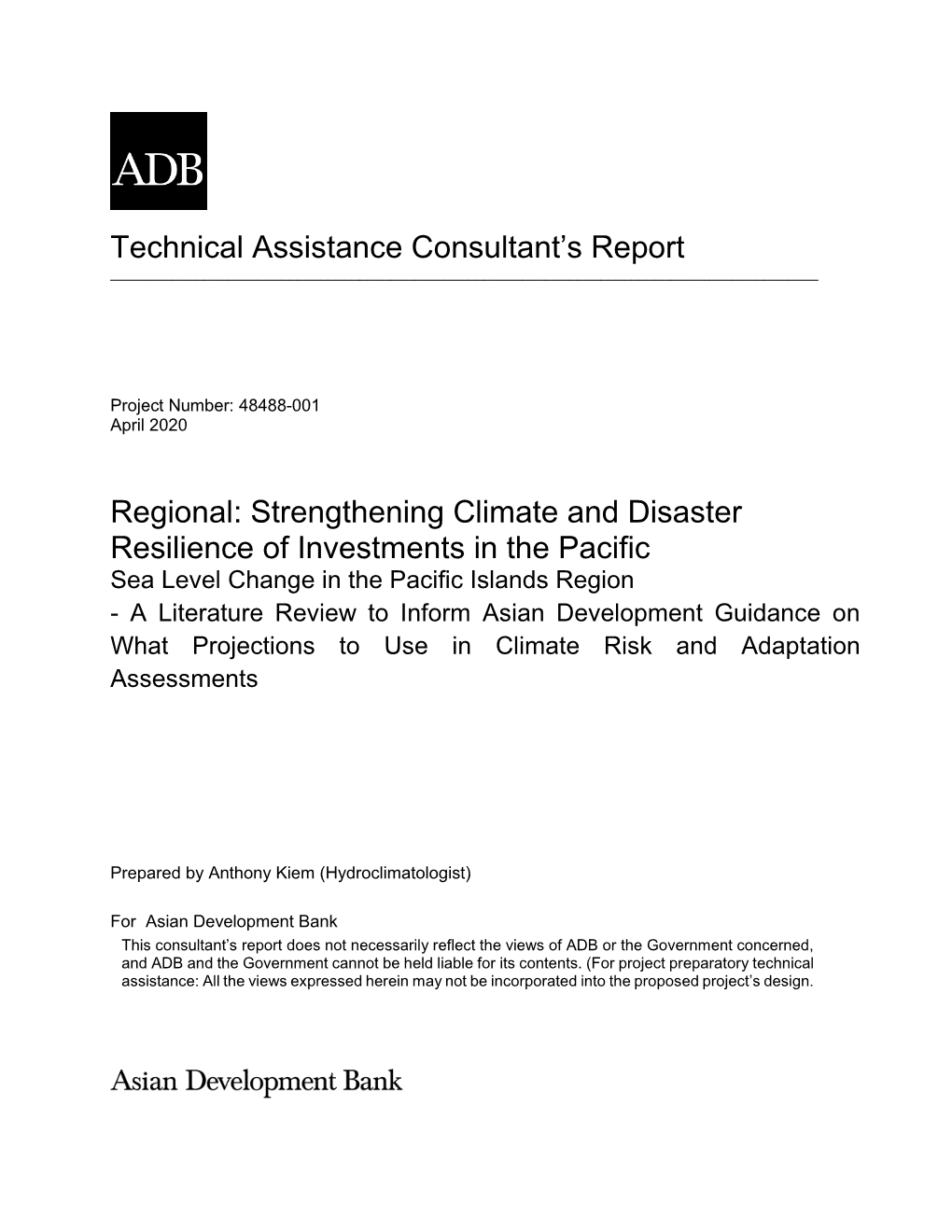
Load more
Recommended publications
-
Human Activities and Flood Hazards and Risks in the South West Pacific: a Case Study of the Navua Catchment Area, Fiji Islands
HUMAN ACTIVITIES AND FLOOD HAZARDS AND RISKS IN THE SOUTH WEST PACIFIC: A CASE STUDY OF THE NAVUA CATCHMENT AREA, FIJI ISLANDS Katalaine Duaibe A thesis submitted to Victoria University of Wellington in partial fulfillment of the requirements for the degree of Master in Science in Physical Geography School of Geography, Environment and Earth Science Victoria University of Wellington 2008 Abstract Human activity is increasingly becoming a factor contributing to ‘disasters’ that occur worldwide. As evident in the Asian Tsunami of 2004, the high levels of loss of life and livelihood, and damage to property were largely due to the population density and human development of the physical landscape of the stricken region. The magnitude of natural hazards coupled with the high population density and low levels of development can have disastrous or catastrophic impacts on a nation as a whole, especially in small island states. Furthermore, the lack of governance structures, legislative compliance, and regulatory land use and planning coupled with the perception of risk of the general public, can all contribute to the magnitude of disasters. The flood plains of Navua, a small rural town outside of Fiji’s capital and prone to all types of flooding, are explored to determine the extent to which human activities impact on the magnitude of flooding and flood damage. Human activities such as land degradation, deforestation of catchment areas, increased population density along the Navua riverbanks inadequate land use planning, zoning, and control of flood plain development; and inadequate drainage, and management of discharges are examined when determining the factors that have contributed to the increased incidence of flooding of the past 100 years until 2004. -
1.1 Fiji Humanitarian Background
1.1 Fiji Humanitarian Background Fiji Humanitarian Background Calamities and Seasonal Affects Capacity and Contacts for In-Country Emergency Response Fiji Humanitarian Background Disasters, Conflicts and Migration Nat Y Comments / Details ura e l s Dis / ast No ers Dr Y The areas affected by drought in Fiji are mainly the smaller islands and the Western and Northern sides of the main islands. Droughts which ou es occur in Fiji are linked to the El Nino Southern Oscillation (ENSO) and associated reductions in rainfall. ght Ear Y Fiji is exposed to earthquakes. Those areas having the greatest earthquake activity are the Taveuni- Udu area, the south western parts of thq es Kadavu and the northern Yasawas. The medium activity extends from the southern part of Taveuni and Vanua Levu, through Koro islands, ua Gau islands, Ovalau, eastern and southern Viti Levu, as far north as north-western Kadavu. The rest of Fiji is a region of relatively low kes earthquake activity. Epi Y Like many developing countries, Fiji is still undergoing an epidemiological transition and is faced with a double burden of communicable and de es non-communicable diseases. Whilst there has been a decline in the incidence of some of the communicable diseases over the past 20 years mi such as tuberculosis and filariasis, the rise in incidence of Leptospirosis and typhoid fever in recent years is a cause for concern. cs Ext No - re me Te mp era tur es Flo Y Flooding is also a significant hazard in Fiji, usually associated with cyclone or tropical depression rainfall. -
Royal Commomwealth Society
THE ROYAL COMMONWEALTH SOCIETY “The Gables”, 15 FINCH ST, EAST MALVERN, VICTORIA , AUSTRALIA Phone: 03 9571 5688 FEBRUARY- MARCH 2019 COMMONWEALTH NEWS A history in common, a future in progress The Royal Commonwealth Society founded in 1868, is constituted by Royal Charter. A self funded, Not for Profit Society committed to improving the lives and prospects of Commonwealth citizens. Through youth empowerment, education and advocacy, the Royal Commonwealth Society promotes the value and the values of the Commonwealth. We champion human rights, democracy and sustainable development across the 53 member states which are intrinsically linked through their common history and shared values. Worldwide Patron: HM the Queen. Victoria Patron: Her Excellency the Honourable Linda Dessau AC, Governor of Victoria 150th Anniversary - 26th June 2018 – London 100th Anniversary – 08th February 2021 – Victoria LUNCHEON: 2019 Calendar of Events Thursday 28th FEBRUARY 2019 Day time rd Luncheon time: 12.00hr for 12.30hr 23 May GUEST Speaker nd 22 August GUEST Speaker th Speaker: Euphemia Harahwa 28 November GUEST Speaker Topic: Emigration from Zimbabwe and the Night Time – Dates. Themes based Challenges of that Journey” around Who we are, what we do and what affects you. th RSVP by WEDNESDAY 20 February 12 FEBRUARY Cancelled. th Thursday 14 March Cost $45 00 Members $55.00 Guests GUEST SPEAKER - the Commonwealth, World Issues , International Peace & Security COMMONWEALTH DAY - DINNER: th 11 April, Thursday 14th MARCH 2019 GUEST SPEAKER - Community, History and Fellowship 13th June, TIME: 630hr – Drinks & Canapés 2 Course Dinner & GUEST SPEAKER - Culture, Human Rights Dessert . Tolerance, Respect And Understanding th 12 September SPEAKER: Mr. -
MASARYK UNIVERSITY BRNO Diploma Thesis
MASARYK UNIVERSITY BRNO FACULTY OF EDUCATION Diploma thesis Brno 2018 Supervisor: Author: doc. Mgr. Martin Adam, Ph.D. Bc. Lukáš Opavský MASARYK UNIVERSITY BRNO FACULTY OF EDUCATION DEPARTMENT OF ENGLISH LANGUAGE AND LITERATURE Presentation Sentences in Wikipedia: FSP Analysis Diploma thesis Brno 2018 Supervisor: Author: doc. Mgr. Martin Adam, Ph.D. Bc. Lukáš Opavský Declaration I declare that I have worked on this thesis independently, using only the primary and secondary sources listed in the bibliography. I agree with the placing of this thesis in the library of the Faculty of Education at the Masaryk University and with the access for academic purposes. Brno, 30th March 2018 …………………………………………. Bc. Lukáš Opavský Acknowledgements I would like to thank my supervisor, doc. Mgr. Martin Adam, Ph.D. for his kind help and constant guidance throughout my work. Bc. Lukáš Opavský OPAVSKÝ, Lukáš. Presentation Sentences in Wikipedia: FSP Analysis; Diploma Thesis. Brno: Masaryk University, Faculty of Education, English Language and Literature Department, 2018. XX p. Supervisor: doc. Mgr. Martin Adam, Ph.D. Annotation The purpose of this thesis is an analysis of a corpus comprising of opening sentences of articles collected from the online encyclopaedia Wikipedia. Four different quality categories from Wikipedia were chosen, from the total amount of eight, to ensure gathering of a representative sample, for each category there are fifty sentences, the total amount of the sentences altogether is, therefore, two hundred. The sentences will be analysed according to the Firabsian theory of functional sentence perspective in order to discriminate differences both between the quality categories and also within the categories. -
South Pacific Newsletter : 15
South Pacific newsletter : 15 著者 "Kagoshima University Research Center for the Pacific Islands" journal or South Pacific newsletter publication title volume 15 page range 1-8 URL http://hdl.handle.net/10232/15801 ISSN 1341-2418 OUTH PACIFIC NEWSLETTER ^i^j> Haval Research Laboratorry http://www.nrlmry.navy.mil/sat_products.html Ir Temperature (Celsius) —> -30 -20 -10 KAGOSHIMA UNIVERSITY RESEARCH CENTER FOR THE PACIFIC ISLANDS CONTENTS The problems oftropical cyclones in the South Pacific 1 Research Seminars 5 Recent Publications 8 Front Page Photo: Tropical Cyclone Ami approaches the Fiji Islands on 12th January 2003. Infra-red satellite image, US Naval Research Laboratory, (http ://www.nrlmry.navy.mil/sat_products.html) The problems of tropical cyclones in the South Pacific James P. Terry Research Center for the Pacific Islands, Kagoshima University (Geography Department, The University ofthe South Pacific) Tropical cyclone climatology The most extreme meteorological conditions in the South Pacific ocean occur during tropical cyclones and island nations are vulnerable to their effects. A tropical cyclone is an intense depression or low pressure system that develops over a large mass of warm ocean water (>26°C), organised in bands of cloud rotating clockwise in the southern hemisphere. Violent winds circulate around a central eye of calm weather, where atmospheric pressure at sea-level may drop below 930 millibars (Fig. 1). Tropical cyclones are relatively infrequent events in the south west Pacific with an average of approximately ten events per season (Table 1). They are often associated with the El Nino/Southern Oscillation (ENSO) phenomenon. At the start of El Nino events, convective storms and tropical cyclones may be generated as the eastward-migrating pool of warm ocean water passes across the north of the South Pacific islands. -
Effects of Climate Change on Settlements and Infrastructure Relevant to the Pacific Islands
PACIFIC MARINE CLIMATE CHANGE REPORT CARD Science Review 2018: pp 159-176 Effects of Climate Change on Settlements and Infrastructure Relevant to the Pacific Islands John Connell, University of Sydney, Australia. EXECUTIVE SUMMARY From around the time of independence, mostly in the 1970s, the coastal zones of most Pacific states have experienced changes in land use, from being primarily agricultural to becoming increasingly urban and tourism orientated. This ‘coastal squeeze’ has led to a concentration of infrastructure and wealth in low lying coastal areas, prone to multiple natural hazards, the impacts of which are exacerbated by climate change. Climate change projections for the Pacific suggest that sea levels will rise by as much as 1.4 m by the end of the century potentially increasing erosion and coastal flooding brought about by storm surges and cyclones superimposed on rising sea levels. Cyclones are likely to be less frequent but more severe and over an expanded area, evident in the recent impacts of Cyclones Pam and Winston. Changes in temperature and rainfall indicate a general warming and drying trend, variable across the region, with a probable greater incidence of drought in association with El Niño Southern Oscillation (ENSO) events, but also an increased frequency and severity of pluvial flooding. These physical changes are likely to lead to increased threats to coastal population concentrations, especially urban settlements, with accompanying negative impacts on national economies. The impacts are and will be greatest for atolls and atoll states. Introduction Climate change is of considerable significance in the surface temperatures (projected to increase by up to Pacific region. -
Hydrological Responses in the Fiji Islands to Climate Variability and Severe Meteorological Events
Regional Hydrological Impacts of Climatic Change—Hydroclimatic Variability (Proceedings of symposium S6 held during the Seventh IAHS Scientific Assembly at Foz do Iguaçu, Brazil, 33 April 2005). IAHS Publ. 296, 2005. Hazard warning! Hydrological responses in the Fiji Islands to climate variability and severe meteorological events JAMES TERRY Geography Department, The University of the South Pacific, PO Box 1168, Suva, Fiji Islands [email protected] Abstract Extreme meteorological events and their hydrological consequences create hardship for developing nations in the South Pacific. In the Fiji Islands, severe river floods are generated by tropical cyclones during the summer wet season, causing widespread devastation and threatening food security. In January 2003, Hurricane Ami delivered torrential rainfalls and record- breaking floods, owing to the rapid approach of the storm, the steep volcanic topography promoting strong orographic effects and hydrological short- circuiting, and landslides temporarily damming tributary channels then subsequently failing. At the opposite extreme, hydrological droughts are experienced during prolonged El Niño phases. Fiji’s population relies on streams for water resources, so episodes of dry season rain failure and low flows over recent decades caused severe water crises. Low discharges in the major Ba River show good correspondence with the SOI, although minimum Q lags behind lowest SOI values by several weeks. Regional ocean warming and more sustained El Niño-like conditions in future may increase the recurrence and intensity of flood-and-drought cycles. Key words El Niño; Fiji Islands; floods; hydrological droughts; tropical cyclones INTRODUCTION For the volcanic Fiji Islands in the tropical southwest Pacific, extreme high and low river flows, i.e. -
Fiji and Disasters References by Kirstie Méheux [email protected] 16 September 2007 Available From
Fiji and Disasters References By Kirstie Méheux [email protected] 16 September 2007 Available from http://www.islandvulnerability.org/fijirefs.pdf This is a list of texts which I have come across in the course of my PhD research (see http://www.islandvulnerability.org/fiji.html#meheuxphd ). The texts are predominantly related to disasters in Fiji, however, there are a few which have a broader Pacific-wide focus. I have also included a few texts that focus on climate change in the Pacific. Benson, C. (1997) The economic impact of natural disasters in the Fiji. Overseas Development Institute Working Paper, Working Paper 97, Overseas Development Institute, London. Brookfield, H. C. (1977) The Hurricane Hazard Natural disaster and small populations. UNESCO/UNFPA MAB (Series: Population and Environment Project in the Eastern Islands of Fiji). Campbell, J. (1994) Disaster Losses in the Pacific: Are They Increasing? In, The South Pacific Regional Disaster Housing Conference held Lae, Papua New Guinea, Campbell, J. (1999) Pacific Island vulnerabilities towards the end of the twentieth century. In, Ingleton, J. (Ed.) Natural Disaster Management: A presentation to commemorate the International Decade for Natural Disaster Reduction (IDNDR) 1990-2000, Tudor Rose, Leicester, 320. Campbell, J. R. (1984) Dealing with Disaster: Hurricane Response in Fiji. Pacific Islands Development Program, East-West Centre, Honolulu. Campbell, J. R. (2006) Traditional disaster-reduction in Pacific Island communities. GNS Science Report, 206/038, GNS Science, Campbell, J. R. and Chung, J. (1986) Post-Disaster Assessment: A. Management. East- West Center, Honolulu. Campbell, J. R. and Chung, J. (1986) Post-Disaster Assessment: B. Field Survey. -
Rural and Outer Islands Development Project - Valaga Bay Port - Engineering Assessment
ASIAN DEVELOPMENT BANK ENVIRONMENTAL IMPACT ASSESSMENT (EIA) SAVUSAVU PORT DEVELOPMENT GOVERNMENT OF FIJI January 2008 CURRENCY EQUIVALENTS (as of 24 May 2007 - as per Draft Report) Currency Unit – Fijian Dollars (F$) F$1.00 = US$0.615 US$1.00 = F$1.63 ABBREVIATIONS ADB Asian Development Bank AFL Airports Fiji Limited ALP Alternative Livelihoods Project ALTA Agricultural Land Tenure Act ASR Air Sensitive Receiver BQA bi-lateral quarantine agreement BRI Bank Rakyat Indonesia CCSLA Cane-farmers Cooperative Savings and Loan Association CIDA Coconut Industry Development Authority DWT Dead Weight Tonnes DFR draft final report DNR Department of National Roads EIRR economic internal rate of return EU European Union F$ Fiji Dollars FAO Food and Agriculture Organisation of the United Nations FCA Fiji College of Agriculture FCOSS Fiji Council of Social Services FDB Fiji Development Bank FEA Fiji Electricity Authority FIMSA Fiji Islands Maritime Safety Administration FIT Fiji Institute of Technology FPCL Fiji Ports Corporation Limited FR final report FRUP Fiji Road Upgrading Project (I, II, III and IV) FSC Fiji Sugar Corporation FSCUL Fiji Savings and Credit Union League FVB Fiji Visitors Bureau GDP Gross Domestic Product GSMT qoliqoli (native fishing rights) support management teams HACCP hazard analysis critical control pathway ICT information and communications technology MASLR Ministry of Agriculture, Sugar and Land Resettlement MFNP Ministry of Finance and National Planning MFI Micro Finance Institution MFU Micro Finance Unit of NCSMED MoE -
Themestudy2013-Full2
UNITED NATIONS Economic andESCAP Social Commission for Asia and the Pacic I II FOREWORD Building resilience to multiple shocks is one of the most pressing contemporary development challenges faced by Asia and the Pacific. Economic crises and natural disasters are on the rise and know no boundaries; they straddle wide geographic areas, spread across all sectors of economic activity, and endanger our communities. For communities still living in fragile and conflict-affected States, each shock erodes their capacity to cope with the next disaster or crisis on the horizon. They are twice as likely to be undernourished and their children three times as likely to be out of school, while they receive less than half the amount that Governments in other countries spend on education, health and security. These communities are stuck in life-long vulnerability traps from which it is very difficult to break out. Five years ago, global economies plunged into deep crises as they struggled under the weight of the most severe economic slowdown since the 1930s. In Asia and the Pacific, the financial crisis converged with the food and fuel crises, which compounded the damage inflicted on the livelihoods of millions of people across the region. Furthermore, in the past few years devastating earthquakes, floods, typhoons, droughts and other natural disasters have wreaked havoc throughout the region, causing enormous loss of lives, and widespread damage to livelihoods, property and local economies. In its wake, climate change has the potential to result in even more disasters among our most vulnerable communities. III Increased connectivity and interdependence through trade and financial flows, dense transport networks and speed of communications, while creating unprecedented opportunities, have also amplified the effects of these multiple shocks. -
ICSU ROAP Science Plan – Special
About ICSU ROAP ICSU Regional Office for Asia and the Pacific was inaugurated on the 19 September by the Deputy Prime Minister of Malaysia, Y.A.B. Dato’ Seri Najib Tun Abdul Razak. The new office will promote the development of science throughout Asia and the Pacific and help strengthen the voice of developing countries in this region. It will also ensure that its scientists become involved in those aspects of the ICSU 2006-2011 Strategic Plan that are especially relevant for this area. Mission Statement ICSU mobilizes the knowledge and resources of the international science community for the benefit of society, to: • identify and address major issues of importance to science and society • Facilitate interaction amongst scientists across all disciplines and from all countries • Promote the participation of all scientists in the international scientific endeavour, regardless of face, citizenship, language, political stance or gender • Provide independent, authoritative advice to stimulate constructive dialogue between the scientific community and governments, civil society, and the private sector Suggested citation : International Council for Science Regional Office for Asia and the Pacific (2008) Science Plan on Hazards and Disasters : Special Vulnerability of Islands © ICSU ROAP 2008 SCIENCE PLAN ON HAZARDS AND DISASTERS Special Vulnerability of Islands Report of ICSU ROAP Planning Group on Natural and Human Induced Enviromental Hazards and Disasters June 2008 V PREFACE 2 ACKNOWLEDGEMENTS 2 EXECUTIVE SUMMARY 3 INTRODUCTION TO SPECIAL -
6 Climate Change and Future Flood Risk in Fiji
COSTS AND BENEFITS OF ECOSYSTEM-BASED ADAPTATION FOR FLOOD RISK REDUCTION IN FIJI P. Brown, Landcare Research, [email protected] A. Daigneault, Landcare Research, [email protected] D. Gawith, Oxford University, [email protected] Selected Paper prepared for presentation at the Agricultural & Applied Economics Association’s 2014 AAEA Annual Meeting, Minneapolis, MN, July 27-29, 2014. Copyright 2014 by Brown, Daigneault, and Gawith. All rights reserved. Readers may make verbatim copies of this document for non-commercial purposes by any means, provided that this copyright notice appears on all such copies. Abstract This paper quantifies the economic impacts of flooding in the Ba River and Penang River catchments in Viti Levu, Fiji. We conducted a detailed assessment of flood damage stemming from two major flooding events in 2012 that severely affected the two important catchments, primarily by using evidence from a novel survey administered in early 2013. We combine these empirical measures of damage with GIS data to estimate total damages from flooding and find that the January 2012 flood caused FJ$36.4 and FJ$12.2 in damages for the Ba River and Penang River catchments, respectively, while the March 2012 flood caused FJ$24.1 and FJ$8.4 in damages for the Ba River and Penang River catchments, respectively. We then estimate the cost of future flooding under moderate and severe climate change projections. Flooding is projected to become more frequent and more severe under both scenarios, with annual losses increasing by 100% with moderate climate change and by 300% with severe climate change.