Decameter Structure in Heater‐Induced Airglow at the High Frequency Active Auroral Research Program Facility
Total Page:16
File Type:pdf, Size:1020Kb
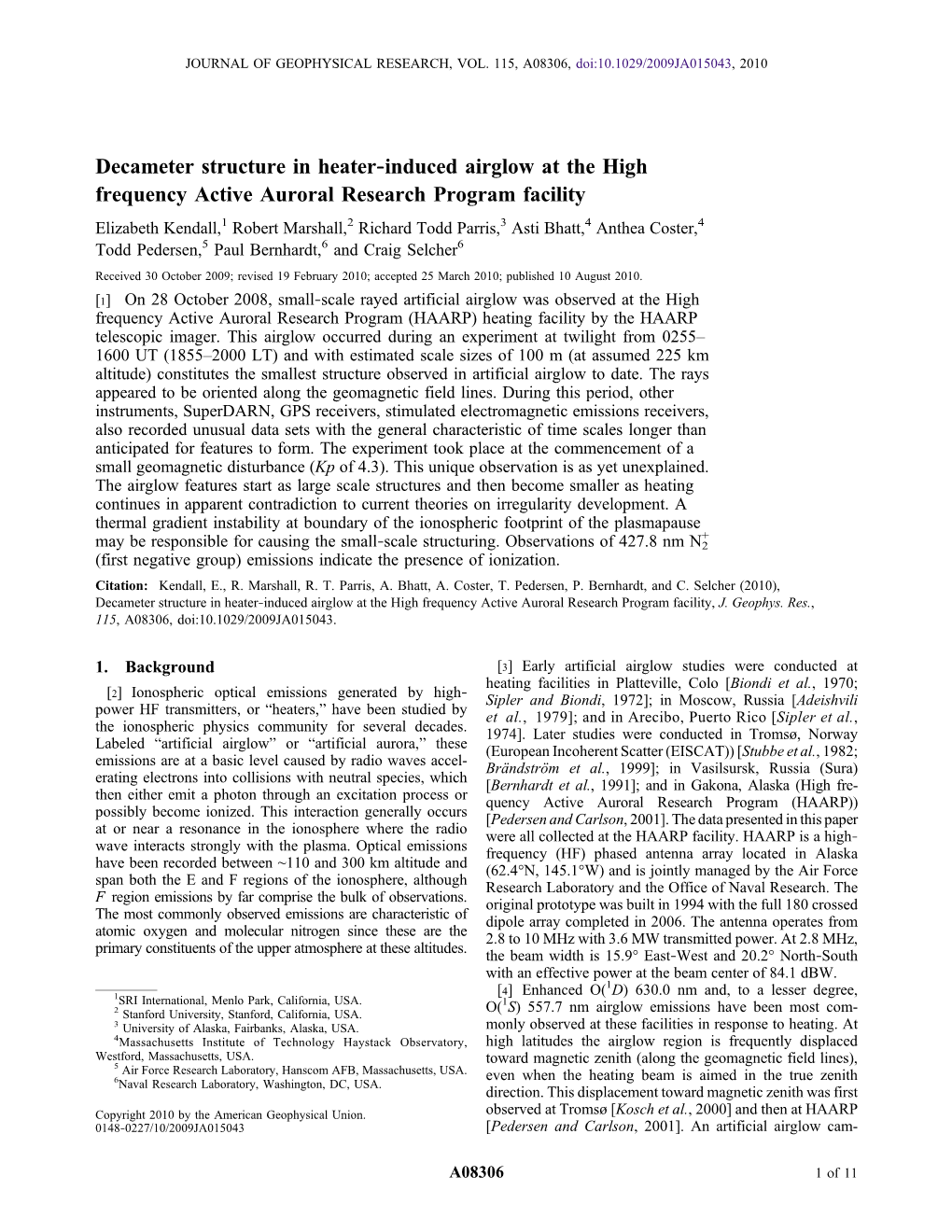
Load more
Recommended publications
-
Abstract a Highly Efficient, Megawatt Class Constant
ABSTRACT Title of dissertation: A HIGHLY EFFICIENT, MEGAWATT CLASS CONSTANT IMPEDANCE TUNABLE POWER EXTRACTION CIRCUIT FOR MOBILE IONOSPHERIC HEATERS Amith Hulikal Narayan Doctor of Philosophy, 2020 Dissertation directed by: Professor Thomas M Antonsen Jr Department of Electrical Engineering Ionospheric modification (IM) refers to changes in the ambient properties of the ionosphere that are produced by humans. The ability to control and exploit iono- spheric processes helps us understand naturally occurring phenomena like aurora, scintillations, and airglow. It also helps us improve trans-ionospheric communica- tion and develop new applications that take advantage of the ionosphere as an active plasma medium. Ionospheric Heaters broadcast high power radio waves, typically in the radio frequency (RF) band, to modify the ionosphere in a controlled manner. These facilities are permanent terrestrial installations and do not presently support study at different latitudes. However, past IM experiments conducted at high lati- tudes across the world indicate a strong dependence of ionospheric processes on the geomagnetic latitude. Mobile Ionospheric Heaters will allow for the first time, quantitative explo- ration of the ionosphere at different geomagnetic latitudes. These mobile structures must be relatively smaller than the existing arrays (small enough to fit on the barge of a ship) and highly efficient at the same time. The size and efficiency of the terres- trial heating units prevent their reuse in mobile structures. These factors motivate the need for developing novel heater units. Our research focused on a new high power, high-efficiency RF source that consists of a gridless tetrode RF tube and a highly efficient power extraction circuit. -
Coordinated Optical and Radar Observations of Ionospheric Pumping for a Frequency Pass Through the Second Electron Gyroharmonic at HAARP M
JOURNAL OF GEOPHYSICAL RESEARCH, VOL. 112, A06325, doi:10.1029/2006JA012146, 2007 Coordinated optical and radar observations of ionospheric pumping for a frequency pass through the second electron gyroharmonic at HAARP M. J. Kosch,1,2,3 T. Pedersen,1 E. Mishin,4 S. Oyama,5,6 J. Hughes,7 A. Senior,2 B. Watkins,5 and B. Bristow5 Received 30 October 2006; revised 20 January 2007; accepted 12 February 2007; published 23 June 2007. [1] On 4 February 2005, the High-frequency Active Auroral Research Program (HAARP) facility was operated in O and X mode while pointing into the magnetic zenith to produce artificial optical emissions in the ionospheric F layer. The pump frequency was set to 2.85 MHz to ensure passing through the second electron gyroharmonic of the decaying ionosphere. Optical recordings at 557.7 and 630 nm were performed simultaneously with the side-viewing high frequency (HF) and colocated ultra high frequency (UHF) ionospheric radars. No X-mode effects were found. For O-mode pumping, when passing from below to above the second gyroharmonic frequency, the optical intensity shows a distinct increase when the plasma frequency passes through the second electron gyroharmonic, while the UHF backscatter changes from persistent to overshoot in character. The optical intensity decreases when pump wave reflection ceases, dropping to zero when upper-hybrid resonance ceases. The HF radar backscatter increases when the upper-hybrid resonance frequency passes from below to above the second gyroharmonic frequency. These observations are consistent with the coexistence of the parametric decay and thermal parametric instabilities above the second gyroharmonic. -
Defense Technical Information Center (DTIC)
Description of document: Defense Technical Information Center (DTIC) computer- generated bibliography from the Technical Report database, subject terms: High-Frequency Active Auroral Research Program or HAARP, 2014 Request date: 30-September-2014 Released date: 03-October-2014 Posted date: 13-July-2015 Source of document: Defense Technical Information Center (DTIC-R) ATTN: FOIA Requester Service Center 8725 John J. Kingman Road, Suite 0944 Ft. Belvoir, VA 22060-6218 The governmentattic.org web site (“the site”) is noncommercial and free to the public. The site and materials made available on the site, such as this file, are for reference only. The governmentattic.org web site and its principals have made every effort to make this information as complete and as accurate as possible, however, there may be mistakes and omissions, both typographical and in content. The governmentattic.org web site and its principals shall have neither liability nor responsibility to any person or entity with respect to any loss or damage caused, or alleged to have been caused, directly or indirectly, by the information provided on the governmentattic.org web site or in this file. The public records published on the site were obtained from government agencies using proper legal channels. Each document is identified as to the source. Any concerns about the contents of the site should be directed to the agency originating the document in question. GovernmentAttic.org is not responsible for the contents of documents published on the website. DEFENSE TECHNICA L INFORMATION CENTER 8725 JOHN J. KINGMAN ROAD FORT BELVO IR, VIRG INIA 22016-6218 LNRO"l.T' DTIC-R (FOIA 2015-1) OCT 3 2014 RlftRTO Thi is in re pon e to your ema il dated September 30, 2014, received in this office October I, 2014, reque ting information under the Freedom of Information Act (FOIA) (enclosure I). -
Artificial Aurora and Ionospheric Heating by HAARP
Advances in Applied Physics, Vol. 4, 2016, no. 1, 23 - 30 HIKARI Ltd, www.m-hikari.com http://dx.doi.org/10.12988/aap.2016.667 Artificial Aurora and Ionospheric Heating by HAARP S. Hadavandkhani Department of Physics, Islamic Azad University(IAU) Varamin Pishva Branch, Iran Bijan Nikouravan1 Department of Physics, Islamic Azad University (IAU) Varamin Pishva Branch, Iran & The Indian Planetary Society (IPS) Mumbai, 400092, India F. Ghazimaghrebi Department of Physics, Islamic Azad University (IAU) Varamin Pishva Branch, Iran Copyright c 2016 S. Hadavandkhani, Bijan Nikouravan and F. Ghazimaghrebi. This article is distributed under the Creative Commons Attribution License, which permits un- restricted use, distribution, and reproduction in any medium, provided the original work is properly cited. Abstract A recent experiment was achieved at HAARP to study the scaling of the ionospherically generated ELF signal with power transmitted from the high frequency (HF) array. The results were in excellent agreement with computer simulations. The outcomes approving that the ELF power increases with the square of the incident HF power. This paper present a review on the situation of the ionized particles in Ionospheric layer when stimulated by artificial an ELF and VLF external high energy radio waves. Keywords: Ionospheric modification, Ionospheric heating 1Corresponding author 24 S. Hadavandkhani, Bijan Nikouravan and F. Ghazimaghrebi 1 Introduction Near the ground the air is almost unionized and its electrical conductivity is negligibly small. In very near equilibrium atmosphere, gravity force has a powerful controlling effect up to about 1000 km from the ground [1]. The gradient of the refractive index is responsible for the bending of the propagation direction of the electromagnetic wave [2]. -
An Ionospheric Modification Facility for the Magnetic Equator J
An Ionospheric Modification Facility for the Magnetic Equator J. L. Chau, Jicamarca Radio Observatory, Peru ([email protected]) S. A. Gonzalez, Arecibo Observatory, Arecibo, Puerto Rico, USA ([email protected]) M. P. Sulzer, Arecibo Observatory, Arecibo, Puerto Rico, USA ([email protected]) E. Nossa, Cornell University, Ithaca, New York, USA ([email protected]) B. Isham, Interamerican University, Bayamón, Puerto Rico, USA ([email protected]) Summary This is a conceptual proposal for the deployment of an ionospheric modification facility, also called and HF facility or ionospheric heater, near the geomagnetic equator. A heater is a powerful high- frequency (HF), or shortwave, radio transmitter that can be used to induce a number of phenomena in the ionospheric plasma. Some of these phenomena provide insights into complicated plasma physics processes that may occur elsewhere in nature but that are difficult or impossible to explore in the laboratory or numerically. Other HF-induced phenomena provide diagnostics of naturally- occurring ionospheric phenomena. HF ionospheric modification may affect the propagation of radio signals passing through the modified region, which is how the phenomenon was first discovered (i.e. the Radio Luxembourg effect [Bailey and Martyn, 1934]). HF transmissions may modify plasma density, increase electron and ion temperatures (which is why they are called heaters), create field-aligned plasma density irregularities that may be interrogated by small coherent scatter radars, and generate VLF and ELF radiation, which has practical societal utility (e.g. in underwater communications and underground remote sensing). By inducing turbulence, and through other processes, they add energy to electrostatic ion-acoustic and Langmuir modes that may be observed by incoherent scatter. -
EISCAT Scientific Association
The EISCAT_3D Science Case Anders Tjulin1 ([email protected]), Ingrid Mann1,2, Ian McCrea3, Anita Aikio4 and Thomas Ulich5 1EISCAT Scientific Association, Sweden; 2Umeå University, Sweden; 3STFC Rutherford Appleton Laboratory, United Kingdom; 4University of Oulu, Finland; 5Sodankylä Geophysical Observatory, Finland EISCAT_3D EISCAT, with international partners, is preparing to construct the next generation radar: EISCAT_3D. It will consist of multiple phased arrays and use state-of-the-art signal processing and beam-forming techniques to become the world's most sophisticated research radar. The five key capabilities of EISCAT_3D are: ● e Resolution of space-time ambiguity. s a c ● - 3D volumetric capability. e c n ● e EISCAT Scientific Association Sub-beam width measurements. i c EISCAT is an international research organisation, conducting s ● / Continuous monitoring of solar variability on terrestrial 7 fundamental research into solar-terrestrial physics and p atmosphere and climate. f / atmospheric science. t c ● e Model validation for space weather and global change. j EISCAT was founded in 1976 and the first EISCAT data were o r obtained in 1981. This is a unique set of capabilities of one single facility. p / e s EISCAT operates three incoherent scatter radar systems (224 MHz, . d 3 500 MHz, 931 MHz) in northern Fenno-Scandinavia and on t a c Svalbard. s i e . The EISCAT systems are located in Tromsø (Norway), Kiruna w (Sweden), Sodankylä (Finland) and Longyearbyen (Svalbard). w w EISCAT also operates an ionospheric heater and supporting Suggested EISCAT_3D site set-up instruments such as dynasondes. The planned time-line for the EISCAT_3D system is: EISCAT is at the present funded by seven The EISCAT_3D Science Case member organisations from six countries: 2005 – 2009 FP6 Design Study (completed) The EISCAT_3D Science Case is prepared as part of the ● 2010 – 2014 FP7 Preparatory Phase (ongoing) Suomen Akatemia, Finland Preparatory Phase. -
Past, Present and Future of Active Radio Frequency Experiments in Space A
Past, Present and Future of Active Radio Frequency Experiments in Space A. V. Streltsov, Jean-Jacques Berthelier, A. A. Chernyshov, V. L. Frolov, F. Honary, M. J. Kosch, R. P. Mccoy, E. V. Mishin, M. T. Rietveld To cite this version: A. V. Streltsov, Jean-Jacques Berthelier, A. A. Chernyshov, V. L. Frolov, F. Honary, et al.. Past, Present and Future of Active Radio Frequency Experiments in Space. Space Science Reviews, Springer Verlag, 2018, 214 (8), pp.118. 10.1007/s11214-018-0549-7. insu-01916927 HAL Id: insu-01916927 https://hal-insu.archives-ouvertes.fr/insu-01916927 Submitted on 11 Mar 2019 HAL is a multi-disciplinary open access L’archive ouverte pluridisciplinaire HAL, est archive for the deposit and dissemination of sci- destinée au dépôt et à la diffusion de documents entific research documents, whether they are pub- scientifiques de niveau recherche, publiés ou non, lished or not. The documents may come from émanant des établissements d’enseignement et de teaching and research institutions in France or recherche français ou étrangers, des laboratoires abroad, or from public or private research centers. publics ou privés. PAST, PRESENT AND FUTURE OF ACTIVE RADIO FREQUENCY EXPERIMENTS IN SPACE A. V. Streltsov1,2, J.-J. Berthelier3, A. A. Chernyshov4, V. L. Frolov5,6, F. Honary7, M. J. Kosch7,8,9, R. P. McCoy10, E. V. Mishin11, M. T. Rietveld12,13 1Embry-Riddle Aeronautical University, Daytona Beach, Florida, USA; 2National Academy of Sciences at Space Vehicles Directorate, Air Force Research Laboratory, Albuquerque, New Mexico, -
NATIONAL ACADEMIES of SCIENCES and ENGINEERING NATIONAL RESEARCH COUNCIL of the UNITED STATES of AMERICA UNITED STATES NATIONAL
i NATIONAL ACADEMIES OF SCIENCES AND ENGINEERING NATIONAL RESEARCH COUNCIL of the UNITED STATES OF AMERICA UNITED STATES NATIONAL COMMITTEE International Union of Radio Science National Radio Science Meeting 5 - 8 January 2005 Sponsored by USNC/URSI University of Colorado at Boulder Boulder, Colorado USA United States National Committee International Union of Radio Science ABSTRACTS National Radio Science Meeting 5-8 January 2005 University of Colorado, Boulder Sponsored by USNC/URSI Table of Contents Session Page No. Session Page No. Membership Information ..... iii F/Jl .................... 207 Description of URSI ......... iv Gl ..................... 219 Plenary Session ............. G2 ..................... 229 Al ....................... 1 G3 ..................... 241 A2 ...................... 13 G4 ..................... 253 Bl ...................... 19 Hl ..................... 263 B2 ...................... 29 H2 ..................... 271 B3 ...................... 39 H3 ..................... 279 B4 ...................... 49 H4 ..................... 287 BS ...................... 57 HS ..................... 295 B6 ...................... 65 H/Gl ................... 305 B7 ...................... 73 H/G2 ................... 313 BS ...................... 83 H/G3 ........... : ....... 321 B9 ...................... 97 H/G4 ................... 333 B/Dl ................... 107 H/GS ................... 343 Cl ..................... 113 H/G6 ................... 349 Dl ..................... 119 H/G7 ................... 361 D2 .................... -
ESTABLISHING a SPACE PHYSICS and RADIO SCIENCE OBSERVATORY at Gakona, Alaska the Case for ESTABLISHING a SPACE PHYSICS and RADIO SCIENCE OBSERVATORY at Gakona, Alaska
UAF Photo by Todd Paris The case for ESTABLISHING A SPACE PHYSICS AND RADIO SCIENCE OBSERVATORY at Gakona, Alaska The case for ESTABLISHING A SPACE PHYSICS AND RADIO SCIENCE OBSERVATORY at Gakona, Alaska This report describes the existing High Frequency Active Auroral Research Program (HAARP) facilities and the vision for applying them. It makes a case for establishing the observatory by describing the unique and highly valuable new research it will enable. The HAARP facilities, located in Gakona, Alaska, were operated by the Air Force Research Laboratory (AFRL) from 1993 until 2014. The facilities consist of a large high-power (3.6 MW) high-frequency radio transmitter and associated diagnostic instruments used for ionospheric modification experiments. After discontinuing the HAARP program, the Air Force donated the HAARP equipment to the University of Alaska Fairbanks (UAF). Now in possession of these world-leading facilities, UAF is working toward establishing an observatory centered on their unique capabilities. The observatory will focus on RF ionospheric heating, Radio Science, Aeronomy, and Magnetospheric Physics. It will bring together a broad range of instrumentation to function as a year-round observatory. By applying ionospheric modification to the problems of Aeronomy and Magnetospheric Physics, the controlled experimentation techniques of laboratory science will be brought to bear on study of the natural near-earth space environment. Gakona is located in south central Alaska at 62°23’ (63.44° magnetic) North Latitude and 145°09’ West Longitude, about 2.5° south of Fairbanks, which places it in the sub-auroral region most of the time. The location has proven to be fruitful for ionospheric modification studies of the generation of ELF and VLF radio emissions by modulating the auroral electrojet, and for studies of the injection of these waves into the magnetosphere to observe their interaction with radiation-belt energetic particles. -
Enhanced Radar Backscatter from the Ionosphere
Enhanced Radar Backscatter from the Ionosphere SCHLATTER NICOLA MANUEL Licentiate Thesis Stockholm, Sweden 2013 TRITA EE 2013-nn ISSN 1653-5146 KTH School of Electrical Engineering ISRN KTH/EE{13/nn{SE SE-100 44 Stockholm ISBN 978-91-7501-627-6 SWEDEN Akademisk avhandling som med tillst˚andav Kungl Tekniska h¨ogskolan framl¨agges till offentlig granskning f¨oravl¨aggande av rymd och plasma fysik Fredag, 22 Febru- ari 2013, klockan 13.15 i Seminarierummet, Teknikringen 31, Kungliga Tekniska h¨ogskolan, Stockholm. © Schlatter Nicola Manuel, Februar 2013 Tryck: Universitetsservice US AB iii Abstract Incoherent scatter radars are powerful ground based instruments for ionospheric measurements. By analysis of the Doppler shifted backscatter spectrum, contain- ing the signature of electrostatic plasma waves, plasma bulk properties are esti- mated. Occasionally, the backscattered radar power is enhanced several orders of magnitude above the thermal backscatter level. These enhancements occur during naturally disturbed ionospheric conditions and in ionospheric modification experi- ments, where a powerful radio wave is incident on the ionospheric plasma. In both of the cases the non-linearity is thought to be turbulence of electrostatic Langmuir waves. The Langmuir turbulence theory and models account for many features of enhanced ionospheric radar backscatter reported on in the literature. During disturbed conditions, with precipitation of auroral electrons, Langmuir turbulence is thought to be driven by a low energy electron beam. Optical and radar obser- vations of naturally enhanced radar backscatter indicate Alfv´enictype of aurora during events reported on in the literature. However, contrasting conclusions have been drawn from optical observations. While some reports suggest that enhanced radar backscatter is observed at the edge of auroral structures others suggest that the enhanced backscatter region and auroral precipitation are co-located. -
EISCAT in the 21 Century a New Operating Agreement & Investment
EISCAT in the 21st Century A New Operating Agreement & Investment Plan PROSPECTUS An Opportunity to join the World’s premier High-Latitude Space Plasma Research Association Recent extensive updating, involving both radar hardware and software, has positioned the EISCAT Scientific Association as a uniquely capable facility to address the priority research topics in high latitude geophysics. Further possibilities exist, not only to extend and maintain this leadership position to enable the Association to address already identified future priority areas, but also to permit the Association to become the premier data source for continuous, quality, high latitude data. New and existing Associates are invited to join in exploiting these unique scientific opportunities. EISCAT Futures Core Group Asgeir Brekke, Graham Brooks, Ryochi Fujii, Olav Holt, and Tony van Eyken, With valuable contributions from Denis Alcaydé, Tor Hagfors, John Kelly, Ian McCrea, Herman Opgenoorth, Jürgen Röttger, Mike Ruohoniemi, and the members of the EISCAT Futures Group Presented to the EISCAT Council, June 2003 Vn: 16.06.2003; Page 2 Index Executive Summary Introduction Background The present EISCAT facilities The future evolution of Solar Terrestrial Physics The E’ Proposal The philosophy of E’ E’ science goals E’ development model Role of E’ E’ data synthesis E’ educational opportunities Financing E’ E’ investments E’ cost options E’ recurrent costs E’ prospective partners E’ critical timelines Summary Appendices Appendix 1: Contacts Appendix 2: Acronyms, abbreviations, etc. Appendix 3 Review of the past scientific achievements of EISCAT Appendix 4: Science goals Appendix 5: Technical achievements of EISCAT Appendix 6: MST proposal Appendix 7: Example annual budget Executive Summary The EISCAT Scientific Association has lead the World in ionospheric and atmospheric studies using incoherent scatter for most of its lifetime. -
ANGELS DON't PLAY THIS HAARP Advances in Tesla Technology
ANGELS DON‘T PLAY THIS HAARP Advances in Tesla Technology by Dr. Nick Begich and Jeane Manning 1997 from Environmental Warfare? The U. S. Government has a new ground-based ―Star Wars‖ weapon which is being tested in the remote bush country of Alaska. This new system manipulates the environment in a way which can: Disrupt human mental processes. Jam all global communications systems. Change weather patterns over large areas. Interfere with wildlife migration patterns. Negatively affect your health. Unnaturally impact the Earth‘s upper atmosphere. The U.S. military calls its zapper HAARP (High-frequency Active Auroral Research Project). But this skybuster is not about the Northern Lights. This device will turn on lights never intended to be artificially manipulated. Their first target is the electrojet - a river of electricity that flows thousands of miles through the sky and down into the polar icecap. The electrojet will become a vibrating artificial antenna for sending electromagnetic radiation raining down on the earth. The U.S. military can then ―X-ray‖ the earth and talk to submarines. But there‘s much more they can do with HAARP. This book reveals surprises from secret meetings. PROJECT CENSORED - A prestigious panel of journalists judged HAARP to be in the top ten under-reported news stories of 1994. POPULAR SCIENCE - As a front-cover story, HAARP began to be revealed in September, 1995. This book is the rest of the story. The High-frequency Active Auroral Research Project is The HAARP that Angels Don't Play. About the Authors Dr. Nick Begich (image right) is the eldest son of the late United States Congressman from Alaska, Nick Begich Sr., and political activist Pegge Begich.