Paired Sequence Difference in Ribosomal Rnas: Evolutionary and Phylogenetic Implications’
Total Page:16
File Type:pdf, Size:1020Kb
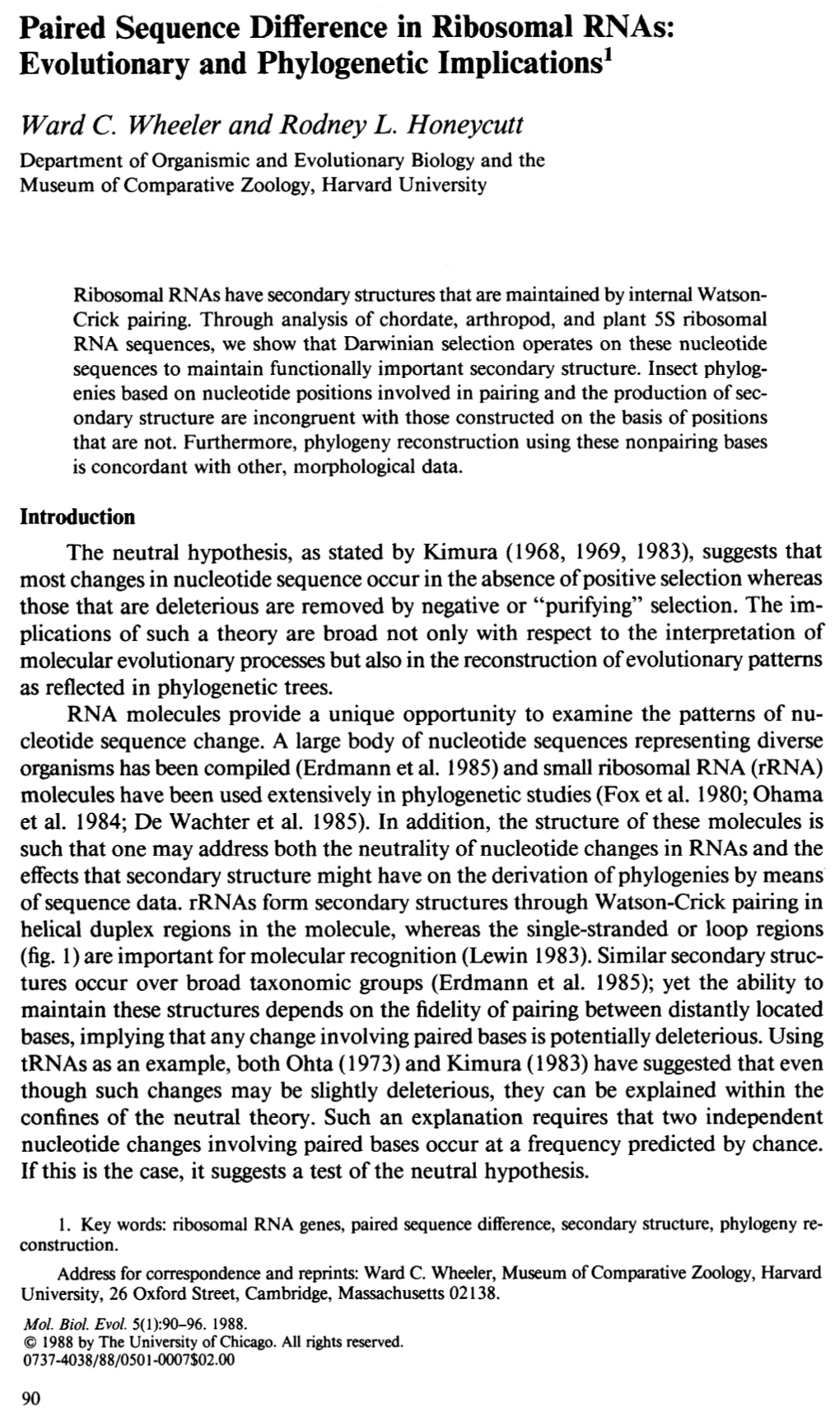
Load more
Recommended publications
-
Analysis of the Complete Mitochondrial DNA Sequence of the Brachiopod Terebratulina Retusa Places Brachiopoda Within the Protostomes
See discussions, stats, and author profiles for this publication at: https://www.researchgate.net/publication/12415870 Analysis of the complete mitochondrial DNA sequence of the brachiopod Terebratulina retusa places Brachiopoda within the protostomes Article in Proceedings of the Royal Society B: Biological Sciences · November 1999 DOI: 10.1098/rspb.1999.0885 · Source: PubMed CITATIONS READS 83 50 2 authors, including: Martin Schlegel University of Leipzig 151 PUBLICATIONS 2,931 CITATIONS SEE PROFILE Some of the authors of this publication are also working on these related projects: Rare for a reason? Scale-dependence of factors influencing rarity and diversity of xylobiont beetles View project Bat diversity and vertical niche activity in the fluvial flood forest Leipzig View project All content following this page was uploaded by Martin Schlegel on 22 May 2014. The user has requested enhancement of the downloaded file. Analysis of the complete mitochondrial DNA sequence of the brachiopod Terebratulina retusa places Brachiopoda within the protostomes Alexandra Stechmann* and Martin Schlegel UniversitÌt Leipzig, Institut fÏr Zoologie/Spezielle Zoologie,Talstr. 33, 04103 Leipzig, Germany Brachiopod phylogeny is still a controversial subject. Analyses using nuclear 18SrRNA and mitochondrial 12SrDNA sequences place them within the protostomes but some recent interpretations of morphological data support a relationship with deuterostomes. In order to investigate brachiopod a¤nities within the metazoa further,we compared the gene arrangement on the brachiopod mitochondrial genome with several metazoan taxa. The complete (15 451bp) mitochondrial DNA (mtDNA) sequence of the articulate brachiopod Terebratulina retusa was determined from two overlapping long polymerase chain reaction products. All the genes are encoded on the same strand and gene order comparisons showed that only one major rearrangement is required to interconvert the T.retusa and Katharina tunicata (Mollusca: Polyplaco- phora) mitochondrial genomes. -
Hemichordate Phylogeny: a Molecular, and Genomic Approach By
Hemichordate Phylogeny: A molecular, and genomic approach by Johanna Taylor Cannon A dissertation submitted to the Graduate Faculty of Auburn University in partial fulfillment of the requirements for the Degree of Doctor of Philosophy Auburn, Alabama May 4, 2014 Keywords: phylogeny, evolution, Hemichordata, bioinformatics, invertebrates Copyright 2014 by Johanna Taylor Cannon Approved by Kenneth M. Halanych, Chair, Professor of Biological Sciences Jason Bond, Associate Professor of Biological Sciences Leslie Goertzen, Associate Professor of Biological Sciences Scott Santos, Associate Professor of Biological Sciences Abstract The phylogenetic relationships within Hemichordata are significant for understanding the evolution of the deuterostomes. Hemichordates possess several important morphological structures in common with chordates, and they have been fixtures in hypotheses on chordate origins for over 100 years. However, current evidence points to a sister relationship between echinoderms and hemichordates, indicating that these chordate-like features were likely present in the last common ancestor of these groups. Therefore, Hemichordata should be highly informative for studying deuterostome character evolution. Despite their importance for understanding the evolution of chordate-like morphological and developmental features, relationships within hemichordates have been poorly studied. At present, Hemichordata is divided into two classes, the solitary, free-living enteropneust worms, and the colonial, tube- dwelling Pterobranchia. The objective of this dissertation is to elucidate the evolutionary relationships of Hemichordata using multiple datasets. Chapter 1 provides an introduction to Hemichordata and outlines the objectives for the dissertation research. Chapter 2 presents a molecular phylogeny of hemichordates based on nuclear ribosomal 18S rDNA and two mitochondrial genes. In this chapter, we suggest that deep-sea family Saxipendiidae is nested within Harrimaniidae, and Torquaratoridae is affiliated with Ptychoderidae. -
Biodiversity
Founded in 1888 as the Marine Biological Laboratory Catalyst SPRING 2012 VOLUME 7, NUMBER 1 IN THIS ISSUE 4 All Species, Great and Small 8 Microbial Diversity, Leaf by Leaf 10 Life, Literature, and the Pursuit of Global Access BIODIVERSITY: Exploring Life on Earth Page 2 F R OM THE D ir ECTO R MBL Catalyst Dear Friends, SPRING 2012 VOLUME 7, NUMBER 1 In February, a group of MBL trustees, overseers, and friends took a memorable MBL Catalyst is published twice yearly by the Office of “eco-expedition” by safari through East Africa. For most of us, the most exciting Communications at the Marine Biological Laboratory aspect was seeing the megafauna – giraffes, zebras, lions, warthogs, wildebeests, (MBL) in Woods Hole, Massachusetts. The MBL is rhinoceroses—roaming wild in a pristine landscape. Except in tropical Asia, these dedicated to scientific discovery and improving the human condition through research and education large, charismatic animals aren’t found anywhere else on the planet—not in the in biology, biomedicine, and environmental science. Americas, Europe, Australia, or New Zealand. Founded in 1888, the MBL is an independent, nonprofit corporation. Why did they disappear? The reasons are debated, but there is good evidence Senior Advisors that overkill by prehistoric humans caused major losses. Unfortunately, the near- President and Director: Gary Borisy Chief Academic and extinction of these species 10,000 to 50,000 years ago is not the end of the story. Scientific Officer: Joshua Hamilton It is generally agreed that the Earth is facing another biodiversity crisis in this Director of External Relations: Pamela Clapp Hinkle century, with extinctions largely driven by destruction of habitat. -
The Fox/Forkhead Transcription Factor Family of the Hemichordate Saccoglossus Kowalevskii
The Fox/Forkhead transcription factor family of the hemichordate Saccoglossus kowalevskii The Harvard community has made this article openly available. Please share how this access benefits you. Your story matters. Citation Fritzenwanker, Jens H, John Gerhart, Robert M Freeman, and Christopher J Lowe. 2014. “The Fox/Forkhead transcription factor family of the hemichordate Saccoglossus kowalevskii.” EvoDevo 5 (1): 17. doi:10.1186/2041-9139-5-17. http://dx.doi.org/10.1186/2041-9139-5-17. Published Version doi:10.1186/2041-9139-5-17 Accessed February 16, 2015 3:46:47 PM EST Citable Link http://nrs.harvard.edu/urn-3:HUL.InstRepos:12717458 Terms of Use This article was downloaded from Harvard University's DASH repository, and is made available under the terms and conditions applicable to Other Posted Material, as set forth at http://nrs.harvard.edu/urn-3:HUL.InstRepos:dash.current.terms- of-use#LAA (Article begins on next page) The Fox/Forkhead transcription factor family of the hemichordate Saccoglossus kowalevskii Fritzenwanker et al. Fritzenwanker et al. EvoDevo 2014, 5:17 http://www.evodevojournal.com/content/5/1/17 Fritzenwanker et al. EvoDevo 2014, 5:17 http://www.evodevojournal.com/content/5/1/17 RESEARCH Open Access The Fox/Forkhead transcription factor family of the hemichordate Saccoglossus kowalevskii Jens H Fritzenwanker1*, John Gerhart2, Robert M Freeman Jr3 and Christopher J Lowe1 Abstract Background: The Fox gene family is a large family of transcription factors that arose early in organismal evolution dating back to at least the common ancestor of metazoans and fungi. They are key components of many gene regulatory networks essential for embryonic development. -
Uncorrected Proof
JrnlID 12526_ArtID 933_Proof# 1 - 07/12/2018 Marine Biodiversity https://doi.org/10.1007/s12526-018-0933-2 1 3 ORIGINAL PAPER 2 4 5 On the larva and the zooid of the pterobranch Rhabdopleura recondita 6 Beli, Cameron and Piraino, 2018 (Hemichordata, Graptolithina) 7 F. Strano1,2 & V. Micaroni3 & E. Beli4,5 & S. Mercurio6 & G. Scarì7 & R. Pennati6 & S. Piraino4,8 8 9 Received: 31 October 2018 /Revised: 29 November 2018 /Accepted: 3 December 2018 10 # Senckenberg Gesellschaft für Naturforschung 2018 11 Abstract 12 Hemichordates (Enteropneusta and Pterobranchia) belong to a small deuterostome invertebrate group that may offer insights on 13 the origin and evolution of the chordate nervous system. Among them, the colonial pterobranchOF Rhabdopleuridae are recognized 14 as living representatives of Graptolithina, a taxon with a rich fossil record. New information is provided here on the substrate 15 selection and the life cycle of Rhabdopleura recondita Beli, Cameron and Piraino, 2018, and for the first time, we describe the 16 nervous system organization of the larva and the adult zooid, as well as the morphological, neuroanatomical and behavioural 17 changes occurring throughout metamorphosis. Immunohistochemical analyses disclosed a centralized nervous system in the 18 sessile adult zooid, characterized by different neuronal subsets with three distinctPRO neurotransmitters, i.e. serotonin, dopamine and 19 RFamide. The peripheral nervous system comprises GABA-, serotonin-, and dopamine-immunoreactive cells. These observa- 20 tions support and integrate previous neuroanatomical findings on the pterobranchD zooid of Cephalodiscus gracilis. Indeed, this is 21 the first evidence of dopamine, RFamide and GABA neurotransmittersE in hemichordates pterobranchs. In contrast, the 22 lecithotrophic larva is characterized by a diffuse basiepidermal plexus of GABAergic cells, coupled with a small group of 23 serotonin-immunoreactive cells localized in the characteristic ventral depression. -
Halogenation Enzymes in Bacteria Associated with the Red-Banded Acorn Worm, Ptychodera Jamaicensis" (2011)
San Jose State University SJSU ScholarWorks Master's Theses Master's Theses and Graduate Research Fall 2011 Halogenation Enzymes in Bacteria Associated with the Red- banded Acorn Worm, Ptychodera jamaicensis Milena May Lilles San Jose State University Follow this and additional works at: https://scholarworks.sjsu.edu/etd_theses Recommended Citation Lilles, Milena May, "Halogenation Enzymes in Bacteria Associated with the Red-banded Acorn Worm, Ptychodera jamaicensis" (2011). Master's Theses. 4099. DOI: https://doi.org/10.31979/etd.snbx-gv6x https://scholarworks.sjsu.edu/etd_theses/4099 This Thesis is brought to you for free and open access by the Master's Theses and Graduate Research at SJSU ScholarWorks. It has been accepted for inclusion in Master's Theses by an authorized administrator of SJSU ScholarWorks. For more information, please contact [email protected]. HALOGENATION ENZYMES IN BACTERIA ASSOCIATED WITH THE RED- BANDED ACORN WORM, PTYCHODERA JAMAICENSIS A Thesis Presented to The Faculty of the Department of Biology San José State University In Partial Fulfillment of the Requirements for the Degree Master of Science by Milena M. Lilles December 2011 © 2011 Milena M. Lilles ALL RIGHTS RESERVED The Designated Thesis Committee Approves the Thesis Titled HALOGENATION ENZYMES IN BACTERIA ASSOCIATED WITH THE RED- BANDED ACORN WORM, PTYCHODERA JAMAICENSIS by Milena M. Lilles APPROVED FOR THE DEPARTMENT OF BIOLOGY SAN JOSÉ STATE UNIVERSITY December 2011 Dr. Sabine Rech Department of Biology Dr. Brandon White Department of Biology Dr. Roy Okuda Department of Chemistry ABSTRACT HALOGENATION ENZYMES IN BACTERIA ASSOCIATED WITH THE RED- BANDED ACORN WORM, PTYCHODERA JAMAICENSIS by Milena M. Lilles Organohalogens have diverse biological functions in the environment. -
Ambulacraria - Andrew B
PHYLOGENETIC TREE OF LIFE – Ambulacraria - Andrew B. Smith AMBULACRARIA Andrew B. Smith Department of Palaeontology, The Natural History Museum, London SW7 5BD, UK Keywords: Echinoderms, crinoids, ophiuroids, asteroids, echinoids, holothurians, hemichordates, enteropneustes, pterobranchs, Xenoturbella, phylogeny, evolution, mode of life, developmental biology, fossil record, biodiversity Contents 1. General Introduction 2. The Echinodermata 2.1. General features 2.2. Crinoidea (sea lillies and feather stars) 2.3. Asteroidea (starfishes and cushion stars) 2.4. Ophiuroidea (brittlestars and basket stars) 2.5. Holothuroidea (sea cucumbers) 2.6. Echinoidea (sea urchins) 2.7. Stem group echinoderms 3. The Hemichordata 3.1. General features 3.2. Pterobranchs 3.3. Enteropneusts. 4. Plactosphaeroidea (Xenoturbella) Bibliography Bibliographical sketch Summary The Ambulacraria is a major clade of invertebrates that are the immediate sister group to the chordates. It comprises the phyla Echinodermata and Hemichordata and the puzzling genus Xenoturbella, the latter being included on the basis of its molecular similarity. The morphological characters uniting Echinodermata and Hemichordata are listed, as are those that characterize each phylum separately. The five classes of echinoderm and two classes of hemichordate are brief described, and information is provided about their mode of life, development, geological history and current views on their phylogenetic relationships. 1. General Introduction Ambulacraria is the formal name applied to a group of invertebrate animals that includes the echinoderms and hemichordates. They are exclusively marine and their origins extend back some 525 Ma to the Cambrian radiation when the earliest examples appear in the fossil record, although their actual origins probably lie slightly earlier, based on molecular clock studies. -
Unexpected Molecular and Morphological Diversity of Hemichordate Larvae from the Neotropics
Received: 18 June 2019 | Accepted: 22 September 2019 DOI: 10.1111/ivb.12273 ORIGINAL ARTICLE Unexpected molecular and morphological diversity of hemichordate larvae from the Neotropics Rachel Collin1 | Dagoberto E. Venera-Pontón1 | Amy C. Driskell2 | Kenneth S. Macdonald III2 | Michael J. Boyle3 1Smithsonian Tropical Research Institute, Balboa, Ancon, Panama Abstract 2Laboratories of Analytical Biology, National The diversity of tropical marine invertebrates is poorly documented, especially Museum of Natural History, Smithsonian those groups for which collecting adults is difficult. We collected the planktonic Institution, Washington, DC, USA 3Smithsonian Marine Station, Fort Pierce, tornaria larvae of hemichordates (acorn worms) to assess their hidden diversity in FL, USA the Neotropics. Larvae were retrieved in plankton tows from waters of the Pacific Correspondence and Caribbean coasts of Panama, followed by DNA barcoding of mitochondrial cy- Rachel Collin, STRI, Unit 9100, Box 0948, tochrome c oxidase subunit I (COI) and 16S ribosomal DNA to estimate their diversity DPO AA 34002, USA. Email: [email protected] in the region. With moderate sampling efforts, we discovered six operational taxo- nomic units (OTUs) in the Bay of Panama on the Pacific coast, in contrast to the single species previously recorded for the entire Tropical Eastern Pacific. We found eight OTUs in Bocas del Toro province on the Caribbean coast, compared to seven spe- cies documented from adults in the entire Caribbean. All OTUs differed from each other and from named acorn worm sequences in GenBank by >10% pairwise distance in COI and >2% in 16S. Two of our OTUs matched 16S hemichordate sequences in GenBank: one was an unidentified or unnamed Balanoglossus from the Caribbean of Panama, and the other was an unidentified ptychoderid larva from the Bahamas. -
Morphogenetic Gradients in Graptolites and Bryozoans
Morphogenetic gradients in graptolites and bryozoans ADAM URBANEK Urbanek, A. 2004. Morphogenetic gradients in graptolites and bryozoans. Acta Palaentologica Polonica 49 (4): 485–504. Despite independent evolution of coloniality in hemichordates and bryozoans, their colonies show common features. In both instances colony is a genet or clonal system composed of zygotic oozooid and a number of blastozooids (= modules) integrated by physical continuity of tissues, sharing a common genotype and subject to common morphogenetic control. In some groups of graptolites and bryozoans, colonies display a regular morphological gradient. Simple graptoloid and bryozoan colonies consist of a proximal zone of astogenetic change and a distal zone of astogenetic repetition. Observed morphological gradient may be attributed to diffusion, along the colony axis, of a morphogen produced by the oozooid; in the zone of astogenetic change the morphogen is above certain threshold level and drops below it in the zone of asto− genetic repetition. This model is supported by observations on regeneration of fractured graptoloid colonies. Regenera− tive branch never displays astogenetic change. The same rule is valid for regeneration of fractured bryozoan colonies. While the early astogeny of simple bryozoan colonies may be explained within the framework of the gradient theory, the late astogeny of more complex ones involves multiple succession of zones of change and repetition, without analogy in astogeny of graptoloids. Thus, late astogeny in bryozoan colonies may be controlled by cyclic somatic/reproductive changes, probably independent of the primary morphogen. Evolutionary changes in the graptoloid colonies involve both the spreading of the novelties over a greater number of zooids (penetrance) and an increase in the degree of phenotypic manifestation of a given character (expressivity). -
Comparative Genomic and Phylogenetic Investigation of the Xenobiotic Metabolizing Arylamine N-Acetyltransferase Enzyme Family
View metadata, citation and similar papers at core.ac.uk brought to you by CORE provided by Elsevier - Publisher Connector FEBS Letters 584 (2010) 3158–3164 journal homepage: www.FEBSLetters.org Comparative genomic and phylogenetic investigation of the xenobiotic metabolizing arylamine N-acetyltransferase enzyme family Anthony E. Glenn a, Eleni P. Karagianni b, Fntigona Ulndreaj b, Sotiria Boukouvala b,* a Toxicology and Mycotoxin Research Unit, Russell Research Center, Agricultural Research Service, United States Department of Agriculture, 950 College Station Road, Athens, GA 30605, USA b Department of Molecular Biology and Genetics, Democritus University of Thrace, Alexandroupolis 68100, Greece article info abstract Article history: Arylamine N-acetyltransferases (NATs) are xenobiotic metabolizing enzymes characterized in sev- Received 12 April 2010 eral bacteria and eukaryotic organisms. We report a comprehensive phylogenetic analysis employ- Revised 24 May 2010 ing an exhaustive dataset of NAT-homologous sequences recovered through inspection of 2445 Accepted 31 May 2010 genomes. We describe the first NAT homologues in viruses, archaea, protists, many fungi and inver- Available online 2 June 2010 tebrates, providing complete annotations in line with the consensus nomenclature. Contrary to the Edited by Takashi Gojobori NAT genes of vertebrates, introns are commonly found within the homologous coding regions of lower eukaryotes. The NATs of fungi and higher animals are distinctly monophyletic, but evidence supports a mixed phylogeny -
Conodonts, Calcichordates and the Origin of Vertebrates
Mitt. Mus. Nat.kd. Berl., Geowiss. Reihe l(1998) 81-92 19.11.1998 Conodonts, Calcichordates and the Origin of Vertebrates Jan Bergstrom ’, Wilfried W. Naumann 2, Jens Viehweg & M6nica Marti-Mus With 6 Figures Abstract Interpretation of early deuterostome evolution and relationships has been hampered by the lack of soft-part preservation in most groups. In addition, a recently revealed upside-down life orientation of vertebrates (the only real notoneuralians) compa- red to other bilateral animals has been misinterpreted as evidence for a unique body design in all deuterostomes, misleading any search for relatives. Regarding echinoderms, the variety of body plans is confusing. The interpretation of some fossils with echinoderm-type calcite skeletons as “calcichordate” ancestors of chordates, however, involves a hypothetical reconstruction of an unusual body plan and a long series of hypothetical transitions. The number of necessary steps is much lower if cephalo- chordates (amphioxus or lancelet) are derived directly from hemichordate enteropneusts. “Sensation interpretations” of fossils (Yunnunozoon, Cuthuymyrus) from Burgess Shale type deposits have added further confusion. Soft-part preservation of cono- dont animals, with V-shaped myomeres and a notochord, shows that they were segmented chordates, while probable eyes and teeth suggest that they were already on the vertebrate side. Key words: Deuterostomes, protostomes, amphioxus, conodont animals, hemichordates, calcichordates, notoneuralians. Zusammenfassung Die Interpretation fruher Deuterostomia hinsichtlich ihrer Evolution und verwandtschaftlichen Beziehungen ist in den meisten Gruppen durch den Mangel an Weichkorpererhaltung sehr erschwert. Die kurzlich entdeckte Tatsache, daB Verte- braten, d. h. die einzigen echten Notoneuralia, im Gegensatz zu anderen bilateral symmetrischen Organismen eine mit ihrer ursprunglichen Oberseite nach unten gerichtete Lebensstellung einnehmen, hat zu der irrtumlichen Ansicht gefuhrt, daB alle Deuterostomia uber einen im Tierreich einzigartigen Bauplan verfugen. -
Distribution of Dehalococcoidia in Marine Sediments and Strategies for Their Enrichment
Distribution of Dehalococcoidia in marine sediments and strategies for their enrichment vorgelegt von Master of Science Camelia Algora geb. in Madrid, Spanien von der Fakultät III – Prozesswissenschaften der Technischen Universität Berlin zur Erlangung des akademischen Grades Doktor der Naturwissenschaften / Doctor rerum naturalium -Dr. rer. nat.- genehmigte Dissertation Promotionsausschuss: Vorsitzender: Prof. Dr. Juri Rappsilber Gutachter: Prof. Dr. Peter Neubauer Gutachter: PD Dr. Lorenz Adrian Gutachter: Prof. Dr. Ricardo Amils Tag der wissenschaftlichen Aussprache: 11. Juli 2016 Berlin 2016 I II “Life on earth is such a good story you cannot afford to miss the beginning. Beneath our superficial differences we are all of us walking communities of bacteria. The world shimmers a pointillist landscape made of tiny living beings” Lynn Margulis Dedicado a mis tíos, Dr. José Luis Gallardo y Dra. María del Carmen López, por plantar la semilla del interés por la Ciencia en mi adolescencia, por su ejemplo y por todo su apoyo III IV DECLARATION OF INDEPENDENT WORK Erklärung zur Dissertation Ehrenwörtliche Erklärung zu meiner Dissertation mit dem Titel: ‘Distribution of Dehalococcoidia in marine sediments and strategies for their enrichment’ hiermit erkläre ich, dass ich die beigefügte Dissertation selbstständig verfasst und keine anderen als die angegebenen Hilfsmittel genutzt habe. Alle wörtlich oder inhaltlich übernommenen Stellen habe ich als solche gekennzeichnet. Wörtlich oder inhaltlich Stellen, die aus meinen persönlichen Publikationen stammen, wurden nicht zusätzlich gekennzeichnet. Ich versichere, dass ich die beigefügte Dissertation nur in diesem und keinem anderen Promotionsverfahren eingereicht habe und dass diesem Promotionsverfahren keine endgültig gescheiterten Promotionsverfahren vorausgegangen sind. _____________________ ______________________________ Ort, Datum Unterschrift V ACKNOWLEDGEMENTS The present work was carried out within the research group of PD Dr.