Ion-Sensitive Field Effect Transistor (ISFET) for MEMS Multisensory Chips at RIT Murat Baylav
Total Page:16
File Type:pdf, Size:1020Kb
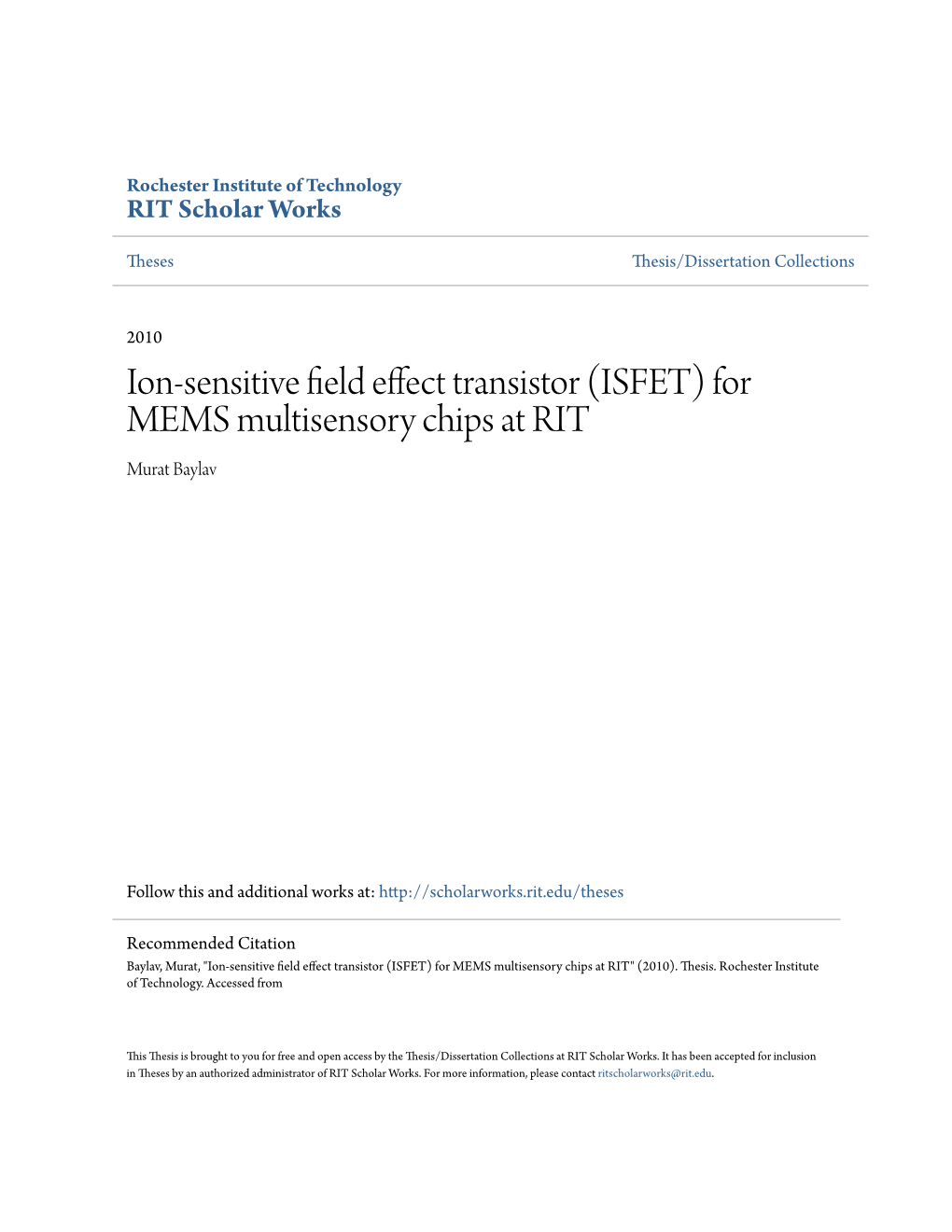
Load more
Recommended publications
-
Temperature Compensation Circuit for ISFET Sensor
Journal of Low Power Electronics and Applications Article Temperature Compensation Circuit for ISFET Sensor Ahmed Gaddour 1,2,* , Wael Dghais 2,3, Belgacem Hamdi 2,3 and Mounir Ben Ali 3,4 1 National Engineering School of Monastir (ENIM), University of Monastir, Monastir 5000, Tunisia 2 Electronics and Microelectronics Laboratory, LR99ES30, Faculty of Sciences of Monastir, University of Monastir, Monastir 5000, Tunisia; [email protected] (W.D.); [email protected] (B.H.) 3 Higher Institute of Applied Sciences and Technology of Sousse (ISSATSo), University of Sousse, Sousse 4003, Tunisia; [email protected] 4 Nanomaterials, Microsystems for Health, Environment and Energy Laboratory, LR16CRMN01, Centre for Research on Microelectronics and Nanotechnology, Sousse 4034, Tunisia * Correspondence: [email protected]; Tel.: +216-50998008 Received: 3 November 2019; Accepted: 21 December 2019; Published: 4 January 2020 Abstract: PH measurements are widely used in agriculture, biomedical engineering, the food industry, environmental studies, etc. Several healthcare and biomedical research studies have reported that all aqueous samples have their pH tested at some point in their lifecycle for evaluation of the diagnosis of diseases or susceptibility, wound healing, cellular internalization, etc. The ion-sensitive field effect transistor (ISFET) is capable of pH measurements. Such use of the ISFET has become popular, as it allows sensing, preprocessing, and computational circuitry to be encapsulated on a single chip, enabling miniaturization and portability. However, the extracted data from the sensor have been affected by the variation of the temperature. This paper presents a new integrated circuit that can enhance the immunity of ion-sensitive field effect transistors (ISFET) against the temperature. -
An Extended CMOS ISFET Model Incorporating the Physical Design
View metadata, citation and similar papers at core.ac.uk brought to you by CORE provided by Spiral - Imperial College Digital Repository IEEE TRANSACTIONS ON ELECTRON DEVICES, VOL. XXX, NO. XXX, XXX XXX 1 An Extended CMOS ISFET Model Incorporating the Physical Design Geometry and the Effects on Performance and Offset Variation Yan Liu, Student Member, IEEE, Pantelis Georgiou, Member, IEEE, Themistoklis Prodromakis, Member, IEEE, Timothy G. Constandinou, Senior Member, and Christofer Toumazou, Fellow, IEEE Abstract—This paper presents an extended model for the as large-scale, highly-integrated chemical sensor arrays [4], CMOS-based Ion-Sensitive-Field-Effect-Transistor (ISFET), in- [6]. corporating design parameters associated with the physical In this paper we present an extended model for CMOS- geometry of the device. This can, for the first time, provide a good match between calculated and measured characteristics by based ISFETs to include both the first order effects, (i.e. taking into account the effects of non-idealities such as threshold intrinsic dimension-related characteristics) and second order voltage variation and sensor noise. The model is evaluated effects, (i.e. non-linear characteristics). By focusing on the through a number of devices with varying design parameters effect of varying the design parameters (i.e physical dimen- (chemical sensing area and MOSFET dimensions) fabricated in sions) a capacitance-based model is derived which includes all a commercially-available 0.35µm CMOS technology. Threshold voltage, subthreshold slope, chemical sensitivity, drift and noise capacitive structures, the values of which are directly related were measured and compared to the simulated results. The to physical dimensions. -
An Integrated Semiconductor Device Enabling Non-Optical Genome Sequencing
ARTICLE doi:10.1038/nature10242 An integrated semiconductor device enabling non-optical genome sequencing Jonathan M. Rothberg1, Wolfgang Hinz1, Todd M. Rearick1, Jonathan Schultz1, William Mileski1, Mel Davey1, John H. Leamon1, Kim Johnson1, Mark J. Milgrew1, Matthew Edwards1, Jeremy Hoon1, Jan F. Simons1, David Marran1, Jason W. Myers1, John F. Davidson1, Annika Branting1, John R. Nobile1, Bernard P. Puc1, David Light1, Travis A. Clark1, Martin Huber1, Jeffrey T. Branciforte1, Isaac B. Stoner1, Simon E. Cawley1, Michael Lyons1, Yutao Fu1, Nils Homer1, Marina Sedova1, Xin Miao1, Brian Reed1, Jeffrey Sabina1, Erika Feierstein1, Michelle Schorn1, Mohammad Alanjary1, Eileen Dimalanta1, Devin Dressman1, Rachel Kasinskas1, Tanya Sokolsky1, Jacqueline A. Fidanza1, Eugeni Namsaraev1, Kevin J. McKernan1, Alan Williams1, G. Thomas Roth1 & James Bustillo1 The seminal importance of DNA sequencing to the life sciences, biotechnology and medicine has driven the search for more scalable and lower-cost solutions. Here we describe a DNA sequencing technology in which scalable, low-cost semiconductor manufacturing techniques are used to make an integrated circuit able to directly perform non-optical DNA sequencing of genomes. Sequence data are obtained by directly sensing the ions produced by template-directed DNA polymerase synthesis using all-natural nucleotides on this massively parallel semiconductor-sensing device or ion chip. The ion chip contains ion-sensitive, field-effect transistor-based sensors in perfect register with 1.2 million wells, which provide confinement and allow parallel, simultaneous detection of independent sequencing reactions. Use of the most widely used technology for constructing integrated circuits, the complementary metal-oxide semiconductor (CMOS) process, allows for low-cost, large-scale production and scaling of the device to higher densities and larger array sizes. -
Dynamic Ph Sensor with Embedded Calibration Scheme by Advanced CMOS Finfet Technology
sensors Article Dynamic pH Sensor with Embedded Calibration Scheme by Advanced CMOS FinFET Technology Chien-Ping Wang 1, Ying-Chun Shen 2, Peng-Chun Liou 1, Yu-Lun Chueh 2, Yue-Der Chih 3, Jonathan Chang 3, Chrong-Jung Lin 1 and Ya-Chin King 1,* 1 Institute of Electronics Engineering, National Tsing Hua University, Hsinchu 30013, Taiwan; [email protected] (C.-P.W.); [email protected] (P.-C.L.); [email protected] (C.-J.L.) 2 Institute of Materials Science and Engineering, National Tsing Hua University, Hsinchu 30013, Taiwan; [email protected] (Y.-C.S.); [email protected] (Y.-L.C.) 3 Design Technology Division, Taiwan Semiconductor Manufacturing Company, Hsinchu 30075, Taiwan; [email protected] (Y.-D.C.); [email protected] (J.C.) * Correspondence: [email protected]; Tel.: +886-3-5162219 or +886-3-5721804 or +1-123914307 Received: 4 March 2019; Accepted: 29 March 2019; Published: 2 April 2019 Abstract: In this work, we present a novel pH sensor using efficient laterally coupled structure enabled by Complementary Metal-Oxide Semiconductor (CMOS) Fin Field-Effect Transistor (FinFET) processes. This new sensor features adjustable sensitivity, wide sensing range, multi-pad sensing capability and compatibility to advanced CMOS technologies. With a self-balanced readout scheme and proposed corresponding circuit, the proposed sensor is found to be easily embedded into integrated circuits (ICs) and expanded into sensors array. To ensure the robustness of this new device, the transient response and noise analysis are performed. In addition, an embedded calibration operation scheme is implemented to prevent the proposed sensing device from the background offset from process variation, providing reliable and stable sensing results. -
A Novel Coding Method for Radar Signal Waveform Design
APPLICATION OF ION SELECTIVE FIELD EFFECT TRANSISTOR FOR HYDROGEN ION MEASUREMENT SYSTEM Ibrahim Elkhier Hussien Arfeen1, Abdul Rasoul Jabar Kizar Alzubaidi2 1 Sudanese Standards and Metrology Organization, Port Sudan 2 Electronic Dept- Engineering College-Sudan University for science and Technology Received December 2010, accepted after revision June 2011 ُمـ ْســتَ ْخـلـَص َهذف هذا انبحث إنً دراصت تزانزصتىر تأثُز انًدبل كًحضبس كًُُبئٍ َضتخذو نًزاقبت اﻷس انهُذروخُنٍ فٍ يدبل انكًُُبء انتحهُهُت و انبُئُت. تعتًذ حضبصُت حضبس تزانزصتىر تأثُز انًدبل عهٍ نىع انًبدة اﻹنكتزونُت انًضتخذيت كًدش فٍ بىابت انتزانزصتىر. وَضتخذو فٍ يىاضع انقُبس انتٍ َصعب فُهب اصتخذاو انًحضبس انزخبخٍ انقببم نهكضز. تضتخذو دائزة إنكتزونُت نزبط يحضبس تزانزصتىر تأثُز انًدبل تًكنه ين انعًم بصىرة فعبنت فٍ انًذي ين اﻷس انهُذروخُنٍ 4 إنً 44. وظُفت انذائزة اﻹنكتزونُت هي تحىَم تُبر دخم انًحضبس انذٌ ًَثم قُى اﻷس انهُذروخنٍ إنً خهذ خزج يتنبصب. تى اختببر انتصًُى بىاصطت يحبنُم قُبصُت وخًضت أنىاع ين انًحبنُم انًبئُت وتى انحصىل عهً اننتبئح انًطهىبت. تى رصى انعﻻقت بُن اننتبئح انًتحصم عهُهب بىاصطت انذائزة اﻹنكتزونُت وقُى اﻷس انهُذروخُنٍ نبعض انًحبنُم انًبئُت. ABSTRACT This paper aims to study field effect transistors as chemical sensor used for monitoring hydrogen ion concentration (H+) measurement in chemical analysis and environment fields, to detect the pH of the subjected solution. The Ion-Selective Field Effect Transistor (ISFET) sensor sensitivity depends mainly on the choice of the gate dielectric material (Silicon Nitride Membrane). The Ion-Selective Field Effect Transistor is (ISFET) used in places where fragile glass electrodes will be damaged. The interface circuit supplies the sensor with a constant current and voltage. The pH value is identified from the output voltage of the circuit. -
Design of Low Noise Ph-ISFET Microsensors and Integrated Suspended Inductors with Increased Quality B
Design of low noise pH-ISFET microsensors and integrated suspended inductors with increased quality B. Palan To cite this version: B. Palan. Design of low noise pH-ISFET microsensors and integrated suspended inductors with increased quality. Micro and nanotechnologies/Microelectronics. Institut National Polytechnique de Grenoble - INPG, 2002. English. tel-00003085 HAL Id: tel-00003085 https://tel.archives-ouvertes.fr/tel-00003085 Submitted on 4 Jul 2003 HAL is a multi-disciplinary open access L’archive ouverte pluridisciplinaire HAL, est archive for the deposit and dissemination of sci- destinée au dépôt et à la diffusion de documents entific research documents, whether they are pub- scientifiques de niveau recherche, publiés ou non, lished or not. The documents may come from émanant des établissements d’enseignement et de teaching and research institutions in France or recherche français ou étrangers, des laboratoires abroad, or from public or private research centers. publics ou privés. ÁÆËÌÁÌÍÌ ÆÌÁÇÆÄ ÈÇÄ Ì ÀÆÁÉÍ Ê ÆÇÄ Ó Æ ØØÖÙ ÔÖ Ð ÐÓØ ÕÙ ÌÀ Ë Æ ÇÌÍÌ ÄÄ ÔÓÙÖ ÓØÒÖ Ð Ö ÇÌÍÊ Ð³ÁÆÈ Ì Ä³ÍÆÁÎÊËÁÌ ÌÀÆÁÉÍ ÌÀÉÍ ÈÊ Í ËÔÐØ ÅÖÓÐØÖÓÒÕÙ ÔÖÔÖ Ù Ð ÓÖØÓÖ ÌÁÅ Ò× Ð Ö Ð³!ÓÐ Ó !ØÓÖÐ ÄÌÊÇÆÁÉ͸ ÄÌÊÇÌÀÆÁÉ͸ ÍÌÇÅ ÌÁÉ͸ ÌÄÇÅÅÍÆÁ ÌÁÇÆË¸ ËÁÆ Ä Ø Ù ÔÖØÑÒØ Ð ÅÖÓÐØÖÓÒÕÙ¸ ÍÒÚÖר ÌÒÕÙ ÌÕÙ ´ÍÌ̵ ÈÖÙ ÔÖ×ÒØ Ø ×ÓÙØÒÙ ÔÙÐÕÙÑÒØ ÔÖ ÓÙ×ÐÚ È Ä Æ Ð ½ÑÖ× ¾¼¼¾ ÌØÖ ÇÆÈÌÁÇÆ ÅÁÊÇ ÈÌÍÊË ÔÀ¹ÁËÌ ÁÄ ÊÍÁÌ Ì ³ÁÆÍÌ ÆË ÁÆÌÊË ËÍËÈÆÍË ÇÊÌ ÌÍÊ ÉÍ ÄÁÌ É ß Ö !Ø ÙÖ× % Ø × & Ó ØÙÖ ÖÒÖ ÇÍÊÌÇÁË ÈÖÓ××ÙÖ ÅÖÓ×ÐÚÀÍËÃ ß ÂÍÊ( ź ÈÖÖ ÆÌÁÄ ¸ÈÖ×ÒØ ź ÖÒÖ ÇÍÊÌÇÁË ¸ÖØÙÖ Ø× ÌÁÅ -
An Ion-Gated Bipolar Amplifier for Ion Sensing with Enhanced Signal and Improved Noise Performance
An ion-gated bipolar amplifier for ion sensing with enhanced signal and improved noise performance Da Zhang, Xindong Gao, Si Chen, Hans Norström, Ulf Smith, Paul Solomon, Shi-Li Zhang, and Zhen Zhang Citation: Applied Physics Letters 105, 082102 (2014); doi: 10.1063/1.4894240 View online: http://dx.doi.org/10.1063/1.4894240 View Table of Contents: http://scitation.aip.org/content/aip/journal/apl/105/8?ver=pdfcov Published by the AIP Publishing Articles you may be interested in Large area graphene ion sensitive field effect transistors with tantalum pentoxide sensing layers for pH measurement at the Nernstian limit Appl. Phys. Lett. 105, 083101 (2014); 10.1063/1.4894078 Hydrogen ion-selective electrolyte-gated organic field-effect transistor for pH sensing Appl. Phys. Lett. 104, 193305 (2014); 10.1063/1.4878539 Nanodiamond-gated silicon ion-sensitive field effect transistor Appl. Phys. Lett. 98, 153507 (2011); 10.1063/1.3568887 Nanodiamond-gated diamond field-effect transistor for chemical sensing using hydrogen-induced transfer doping for channel formation Appl. Phys. Lett. 97, 203503 (2010); 10.1063/1.3518060 Theoretical study of electrolyte gate Al Ga N Ga N field effect transistors J. Appl. Phys. 97, 033703 (2005); 10.1063/1.1847730 This article is copyrighted as indicated in the article. Reuse of AIP content is subject to the terms at: http://scitation.aip.org/termsconditions. Downloaded to IP: 130.238.20.83 On: Wed, 27 Aug 2014 12:08:59 APPLIED PHYSICS LETTERS 105, 082102 (2014) An ion-gated bipolar amplifier for ion sensing with enhanced signal and improved noise performance Da Zhang, Xindong Gao, Si Chen,a) Hans Norstrom,€ Ulf Smith, Paul Solomon,b) Shi-Li Zhang, and Zhen Zhangc) Solid-State Electronics, The A˚ngstrom€ Laboratory, Uppsala University, SE-751 21 Uppsala, Sweden (Received 30 July 2014; accepted 16 August 2014; published online 25 August 2014) This work presents a proof-of-concept ion-sensitive device operating in electrolytes. -
DC I-V Characterization of FET-Based Biosensors Using the 4200A-SCS
DC I-V Characterization of FET-Based Biosensors Using the 4200A-SCS Parameter Analyzer –– APPLICATION NOTE DC I-V Characterization of FET-Based Biosensors APPLICATION NOTE Using the 4200A-SCS Parameter Analyzer Introduction Analyzer shown in Figure 1. This configurable test system simplifies these measurements into one integrated system Extensive research and development have been invested that includes hardware, interactive software, graphics, and in semiconductor-based biosensors because of their low analysis capabilities. cost, rapid response, and accurate detection. In particular, field effect transistor (FET) based biosensors, or bioFETs, This application note describes typical bioFETs, explains are used in a wide variety of applications such as in how to make electrical connections from the SMUs to the biological research, point-of-care diagnostics, environmental device, defines common DC I-V tests and the instruments applications and even in food safety. used to make the measurements, and explains measurement considerations for optimal results. A bioFET converts a biological response to an analyte and converts it into an electrical signal that can be easily measured using DC I-V techniques. The output characteristics (Id-Vd), transfer (Id-Vg) characteristics, and current measurements vs.time (I-t) can be related to the detection and magnitude of the analyte. These DC I-V tests can easily be measured using multiple Source Measure Units (SMUs) depending on the number of terminals on the device. An SMU is an instrument that can source and measure current and voltage and can be used to apply voltage to the gate and drain terminals of the FET. An integrated system that combines multiple SMUs with interactive software is the Keithley 4200A-SCS Parameter Figure 1. -
ISFET Ph Probe Features/Benefits
CS525 ISFET pH Probe Campbell Scientifi c’s CS525 pH probe provides reliable, accurate pH measurements that are fully temperature com- Features/Benefi ts pensated. Th is probe makes general pH measurements in • Innovative ISFET pH-sensing element used that makes aqueous or semi-solid solutions, and can be submersed or better measurements in extreme pH conditions inserted into tanks, pipelines, and open channels. • No clogging or contamination of junction Th e CS525 uses SENTRON’s high-tech, Ion Sensitive Field • Easily cleaned Eff ect Transistor (ISFET) semi-conductor as its pH-sensitive • More rugged than the traditional glass electrode pH element, and includes a silver/silver chloride - potassium probes allowing the CS525 to be deployed in the fi eld chloride reference system. The ISFET technology is for longer time periods the most powerful pH monitoring technology available • Compatible with all Campbell Scientifi c dataloggers1 today. Th is technology considerably reduces the number (including the CR200(X) series) of acidic or alkaline errors in extreme pH conditions. It allows the CS525 to monitor pH in liquids containing high • Designed and manufactured under stringent quality solids, aggressive chemicals, or biological materials that control conditions in an ISO 9001 environment would clog or contaminate the junction of the traditional • Each sensor individually tested glass-bulb pH probes. • CE compliant Th e CS525’s rugged design makes it suitable for just about • Fully temperature compensated any liquid pH-monitoring application, from laboratory to harsh fi eld applications. Th e probe’s electronics are safely embedded in a durable PEEK body. Elimination of the Questions • Research • Pricing glass-bulb removes the possibility of broken glass, making www.campbellsci.com/cs525 the CS525 more durable and safer to use. -
The Mfluence of Optical Radlatlon on the MOSFET Threshold Voltage Has Been Mvestlgated Theoretically As Well As Experimentally F
Sensors and Actuators, 9 (1986) 313 - 321 313 THRESHOLD VOLTAGE VARIATIONS IN N-CHANNEL MOS TRANSISTORS AND MOSFET-BASED SENSORS DUE TO OPTICAL RADIATION W WLODARSKI, P BERGVELD and J A VOORTHUYZEN Department of Electrical Engmeerang, Twente Unwerstty of Technology, P 0 Box 217, 7500 AE Enschede {The Netherlands) (Recewed November 29 1985, m rewsed form February 21, 1986, accepted May 15, 1986) Abstract The mfluence of optical radlatlon on the MOSFET threshold voltage has been mvestlgated theoretically as well as experimentally For conven- tlonal MOSFETs the influence 1s neghglble, but for open-gate FET-based sensors, such as the ISFET, optIcal radiation can cause a considerable threshold voltage shift An explanation of the threshold voltage shrft due to lllummatlon 1s given, based on the analysis of quasi-equlllbnum effects in an lllummated semiconductor surface layer The relation between the threshoid voltage and the optical radiation intensity has been derwed Exper~en~ results are gwen 1 Introduction In recent years, there has been a growmg interest m the utlhzatlon of insulated-gate field-effect transistor structures and related devices as sensors For example, vlslble and mfrared Imagers, prezoelectnc strain sensors, various chemrcal sensors, as well as humldlty and gas sensors based on these structures have all been reported m the literature Although there 1s a vast store of knowledge concerning the effect of optical radiation on the electrophyslcal parameters of MIS capacitors [ 1 - 41, no mformatlon is available pertammg to the -
An Integrated ISFET Ph Microsensor on a CMOS Standard Process
Journal of Sensor Technology, 2013, 3, 57-62 http://dx.doi.org/10.4236/jst.2013.33010 Published Online September 2013 (http://www.scirp.org/journal/jst) An Integrated ISFET pH Microsensor on a CMOS Standard Process Francisco López-Huerta1*, Rosa María Woo-Garcia2, Miguel Lara-Castro1, Johan Jair Estrada-López3, Agustín Leobardo Herrera-May1 1Research Center for Micro and Nanotechnology, University of Veracruz, Veracruz, Mexico 2Faculty of Electronics Sciences, Meritorious Autonomous University of Puebla, Puebla, Mexico 3Faculty of Mathematics, Autonomous University of Yucatán, Mérida, Mexico Email: *[email protected] Received June 7, 2013; revised July 7, 2013; accepted July 15, 2013 Copyright © 2013 Francisco López-Huerta et al. This is an open access article distributed under the Creative Commons Attribution License, which permits unrestricted use, distribution, and reproduction in any medium, provided the original work is properly cited. ABSTRACT We present the design and integration of a nine-pH microsensor array on a single silicon substrate with its own signal readout circuit, integrated in a 0.6-µm commercial standard complementary metal oxide semiconductor (CMOS) proc- ess. An ion sensitive field effect transistor (ISFET) has been used as pH microsensor and an instrumentation amplifier as the read-out circuit. The ISFET structure is conformed by the channel length and ratio of MOS transistor, gate ex- tended and the selective membrane, for which silicon nitride (Si3N4) is employed as an ion selective element. The com- plete design includes shielding around the pH microsensor and the readout circuit to avoid leakage of current to the substrate. The readout circuit is composed by three operational amplifiers and resistances that form the instrumentation amplifier, with a ±2.5 V bias has a 50 dB gain, power supply rejection ratio (PSSR) of 120 dB and common mode re- jection ratio (CMRR) of 127 dB. -
A High Aspect Ratio Fin-Ion Sensitive Field Effect Transistor: Compromises Towards Better Electrochemical Bio-Sensing
A high aspect ratio Fin-Ion Sensitive Field Effect Transistor: compromises towards better electrochemical bio-sensing Serena Rolloᵃ,ᵇ, Dipti Raniᵃ, Renaud Leturcqᵃ, Wouter Olthuisᵇ, César Pascual Garcíaᵃ† a. Materials Research and Technology Department, Luxembourg Institute of Science and Technology (LIST), Belvaux, Luxembourg b. BIOS Lab on Chip Group, MESA+ Institute for Nanotechnology, University of Twente, Enschede, The Netherlands. † Corresponding author: [email protected] . The development of next generation medicines demand more sensitive and reliable label free sensing able to cope with increasing needs of multiplexing and shorter times to results. Field effect transistor-based biosensors emerge as one of the main possible technologies to cover the existing gap. The general trend for the sensors has been miniaturisation with the expectation of improving sensitivity and response time, but presenting issues with reproducibility and noise level. Here we propose a Fin-Field Effect Transistor (FinFET) with a high heigth to width aspect ratio for electrochemical biosensing solving the issue of nanosensors in terms of reproducibility and noise, while keeping the fast response time. We fabricated different devices and characterised their performance with their response to the pH changes that fitted to a Nernst-Poisson model. The experimental data were compared with simulations of devices with different aspect ratio, stablishing an advantage in total signal and linearity for the FinFETs with higher aspect ratio. In addition, these FinFETs promise the optimisation of reliability and efficiency in terms of limits of detection, for which the interplay of the size and geometry of the sensor with the diffusion of the analytes plays a pivotal role.