Active Subaquatic Fault Segments in Lake Iznik Along the Middle Strand
Total Page:16
File Type:pdf, Size:1020Kb
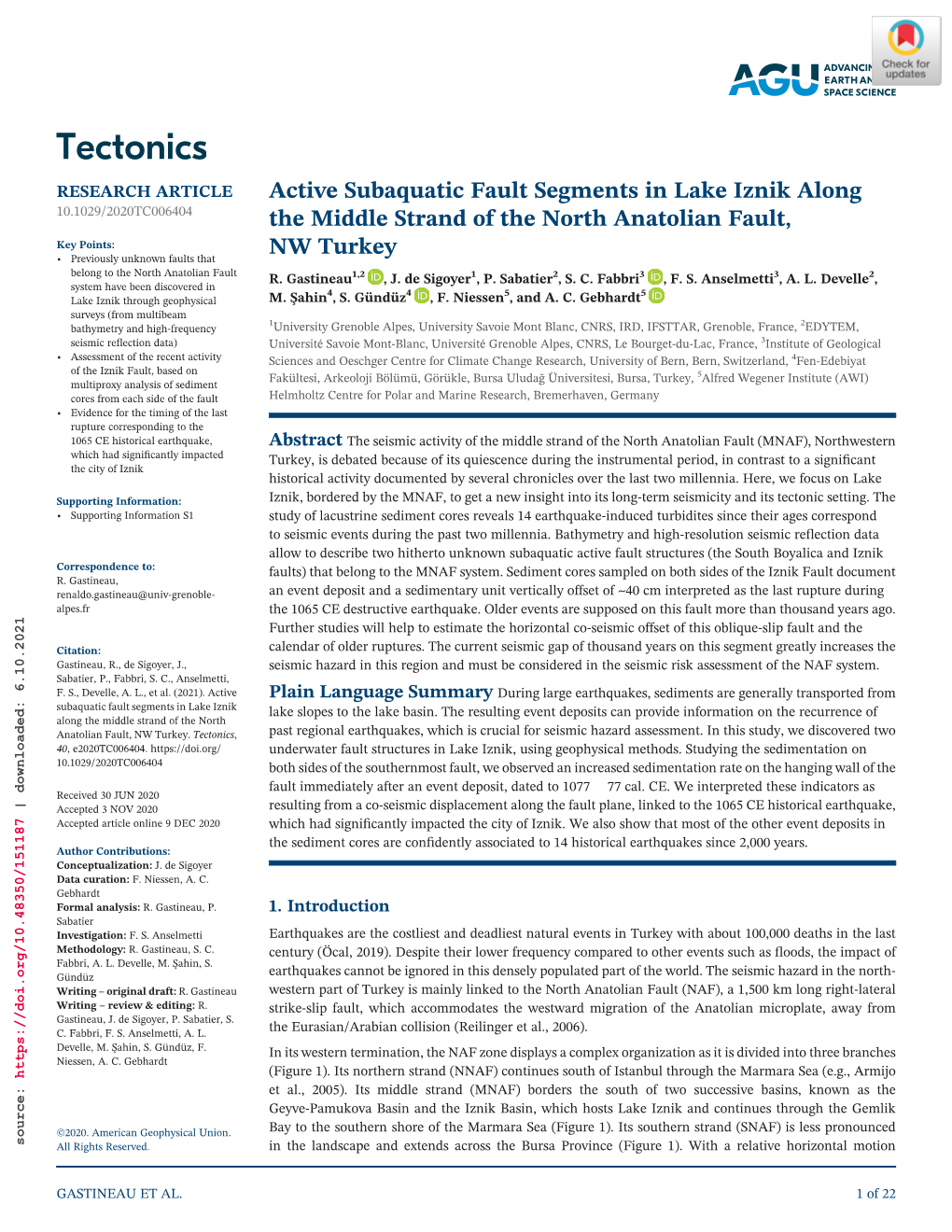
Load more
Recommended publications
-
Some Biological Properties of Carp (Cyprinus Carpio L., 1758) Introduced Into Damsa Dam Lake, Cappadocia Region, Turkey
Pakistan J. Zool., vol. 46(2), pp. 337-346, 2014. Some Biological Properties of Carp (Cyprinus carpio L., 1758) Introduced into Damsa Dam Lake, Cappadocia Region, Turkey Ramazan Mert¹* and Sait Bulut² ¹Department of Biology, Faculty of Arts and Sciences, Nevsehir Hacı Bektaş Veli University, Nevsehir, Turkey. 2Department of Science Education, Faculty of Education, Akdeniz University, Antalya, Turkey. Abstract.- Age composition, length–weight relationships, growth, and condition factors of the carp (Cyprinus carpio L.,1758) were determined using specimens (39.38% female and 60.62% male) collected from Damsa Dam Lake between May 2010 and April 2011. The age composition of the samples was from I to VIII. The length–weight relationship was calculated as W = 0.0181 TL 2.9689 for females and W = 0.0278 TL 2.8507 for males. The total lengths were between 17.1 and 69.2 cm, and the total weights were found to be between 86 and 5473 g. The majority of the individuals (48.12%) were between 46.0 and 55.0 cm length groups. The von Bertalanffy growth equation were found as L∞ = 86.80 cm, K = 0.189, t0 = -0.396 for females and L∞ = 85.34 cm, K = 0.175, t0 = -0.468 for males. The growth performance index was also estimated as Ф′ = 7.260 for females and Ф′ = 7.151 for males. The mean condition factor was found as 1.582 for females and 1.572 for males. The total mortality (Z) was calculated as 0.25 yıl-1. Keywords: Carp, Cyprinus carpio, age composition, condition factor, Damsa Dam Lake. -
Segment Structure of the Southern Strand of the North Anatolian Fault System and Paleoseismic Behaviour of the Gemlik Fault, NW Anatolia
Segment Structure of the Southern Strand of the North Anatolian Fault System and Paleoseismic Behaviour of the Gemlik Fault, NW Anatolia Selim Özalp, Ömer Emre and Ahmet Doğan General Directorate of Mineral Research and Exploration (MTA), Department of Geological Research 06520, Ankara, Turkey The North Anatolian Fault System (NAFS) is a transform fault that accommodates relative motion between Anatolian and Black Sea microplates. The NAFS bifurcates two strands as the Northern and the Southern in the Marmara region. Recent lateral motion is about 24 mm/yr along the NAFS. According to the recent GPS data, there is a slip partitioning between both strands and the northern strand carries approximately 3 times as much right-lateral motion as does the southern strand. Therefore we interpret that the northern strand is the master zone which accommodate majority of the recent lateral motion. In this study, the southern strand is extending between Dokurcun valley and Bandırma bay is evaluated as a splay instead of a main strand diverging from the NAFS as described in previous studies. This splay is included in NW Anatolia transition zone which characterizes bend structures. Length of the southern strand is about 140 km between Dokurcun valley and Gemlik bay along Pamukova basin, Lake İznik and Gemlik Bay depressions. The strand can be divided into three main geometric segments in right steeping pattern. Those are Geyve, İznik and Gemlik, from east to west. Length of the Geyve Fault is about 57 km and general trend is N70°E. The İznik Fault is about 56 km-long trending of N75°E. -
Scanned by Scan2net
Ulud. Uniu. Zir. Fak. Derg. , (1986) 5: 19-26 GENERAL ASPECT OF HORTICULTURE IN BURSA Atilla ERİŞ* Vedat ŞEN1Z * * Arif SOYLU*** SUM MARY Bursa has a special place in Turkey from the horticultural point of uiew. Fruit species such as oliue, peach, grcıp e , apple, plum, pear, cherry, walnut, chest niıt, fig, strawberry and quince and almost all summer and w in ter uegetable specieı that haue important additiues to total production of Turkey are grown in this uicinity extensiuely. Vegetable species such as tomato, bean, pepper and pea whiciı especially processedin industry, and o nion are produced much than others. In this study, current aspect of horticulture in Bursa prouince was identi{ied; and its economic dimensions and technical problems were explained. ÖZET bursa 'da Bahçe Bitkileri Tanınının Genel Durumu Bahçe bitkileri yetiştiriciliğ i açısından Bursa 'nın Türkiye 'de özel bir yeri uar dır. Yörede Türkiye toplam üretimine önemli katkıları olan zeytin, şeftali, üzüm, elmd, erik, armut, kiraz, ceuiz, k eıtan e , incir, çilek ue ayua gibi meyue türleri ile he men hemen tüm yazlık ue kışlık sebze türleri çok yaygın olarak yetiştirilmektedir. Sebzelerden bilha11a sanayide çok iş lene n domates, fasulye, biber, bezelye gibi türler ile soğan · diğ erlerinde n daha da fazla üretilmektedir. Bu çalış mada bahçe bitkilerinin Bursa y ö reıind eki m eucut durumu tan ı tıl mış , ekonomik boyutları ue belirlenen sorunları açıklanmı ş tır. INTRODUCTION It is seldom to find regions which are suitable to horticulture at various alti tutes from sea level up to 2.000 m in the world. Bursa has an important place among these regions having about 1.104.301 ha agricultural area according to the recent statistics (Anonymous 1983 c). -
Seasonal Variations in Zooplankton Species of Lake Gölhisar, a Shallow Lake in Burdur, Turkey
Pakistan J. Zool., vol. 46(4), pp. 927-932, 2014. Seasonal Variations in Zooplankton Species of Lake Gölhisar, a Shallow Lake in Burdur, Turkey Meral Apaydın Yağcı* Fisheries Research Station, 32500, Eğirdir, Isparta, Turkey Abstract.- Seasonal variations of zooplankton species were investigated between Spring 2002 and Winter 2003 in Lake Gölhisar, Burdur, Turkey. A total of 31 species comprising 15 Rotifera (48%), 11 Cladocera (36%), and 5 Copepoda (16%) were recorded. Keratella quadrata, Daphnia longispina and Acanthodiaptomus denticornis were the common species during the study period. Maximum number of taxa were observed from Rotifera and Cladocera during summer, while minimum taxa was determined from Copepoda during winter. Keywords: Rotifera, Cladocera, Copepoda. INTRODUCTION lake Van, (Yildiz et al., 2010), lake Sünnet (Deveci et al., 2011), Beymelek lagoon and lake Kaynak (Yalım et al., 2011), lake İznik (Apaydın Yağcı and In the lake ecosystem, phytoplanktons are Ustaoğlu, 2012). However, the zooplankton fauna of important food source of some invertebrate Lake Gölhisar has not been studied so far. organisms, whereas, zooplanktons provide an The purpose of the investigation was to important food source for larval fish. The major determine the zooplankton species and its seasonal groups of zooplankton in freshwater ecosystems are variations in lake Gölhisar. Rotifera, Cladocera and Copepoda. Many rotifers play an important role in lacustrine food webs MATERIALS AND METHODS because they have a rapid turnover rate and metabolism (Segers, 2004). Rajashekhar et al. Study site (2009) stated that rotifera are sensitive to Lake Gölhisar which is in the western Taurus environmental changes and are therefore useful Mountains in Turkey is established in drainage indicators of water quality. -
Orhangazi İlçe Raporu
ORHANGAZİ Ekim 2012 İçindekiler 1. GENEL GÖRÜNÜM ........................................................................................................................ 3 1.1. Coğrafya ve İklim ......................................................................................................................... 3 1.2. İdari Yapı ....................................................................................................................................... 4 1.3. Tarih ............................................................................................................................................... 5 1.4. Nüfus .............................................................................................................................................. 5 1.5. Sosyal Yapı ................................................................................................................................... 7 1.5.1. Eğitim.................................................................................................................................... 7 1.5.2. Sağlık .................................................................................................................................... 7 2. EKONOMİK GÖRÜNÜM ................................................................................................................ 8 2.1. Genel ............................................................................................................................................. 8 2.2. Tarım ............................................................................................................................................. -
Bursa Ili Maden Ve Enerji Kaynaklari
BURSA İLİ MADEN VE ENERJİ KAYNAKLARI Bursa ili Marmara bölgesinin güneyinde yer alır, sanayi ve ekonomi bakımından Türkiye’nin önemli illerinden biridir. Bursa ilinin arazisi volkanik bir yapıya sahiptir. Türkiye Deprem Haritasına göre, Marmara çevresinde aktif fayların bulunması nedeniyle, Bursa 1. Derece deprem kuşağı içinde yer almaktadır. Bursa ve civarını etkilemesi beklenen sismik faaliyetin kaynağı, Marmara denizindeki faylar, Geyve-İznik fayında Bursa ve civarındaki faylardır. Bursa ilinde küçük fayların yanında, Kuzey Anadolu Fayı ile ilişkili gelişen büyük ölçekli faylar, genç birimleri denetlemektedirler. Bursa ili sosyo-ekonomik bakımdan Türkiye'nin en gelişmiş illerindendir. İlin zengin yer altı kaynakları da sanayisinin gelişmesinde etkili olmuştur. Bursa ilindeki jeolojik çeşitlilik beraberinde birçok cevherleşmeyi de getirmiştir. Genel Müdürlüğümüzün Bursa ili ve yakın çevresinde yaptığı çalışmalar sonucunda önemli endüstriyel hammadde ve metalik maden yatak ve zuhurları ortaya çıkarılmıştır. Metalik madenler bakımından ildeki önemli metalik madenler altın, antimuan, bakır-kurşun- çinko, krom, nikel, manganez, molibden ve volframdır. Bunlardan 3.027 gr/ton Au tenörlü altın İnegöl-Sülüklügöl sahasında tespit edilmiş olup, 19.846 ton görünür+muhtemel, 17.407 ton mümkün rezerv belirlenmiştir. Sahada aynı zamanda %6.5 antimuan tenörlü 14.400 ton antimuan rezervi bulunmaktadır ve yataktan geçmiş yıllarda 1000 ton kadar cevher üretilmiştir. İldeki diğer antimuan cevherleşmeleri Keles ilçesinde gözlenmektedir. Bunlardan % 1.6-2.7 MoS2 tenörlü Kozbudaklar yatağında geçmiş yıllarda bir miktar üretim yapılmıştır. Sahada aynı zamanda % 0.3 WO3 tenörlü 210.00 ton mümkün rezerve sahip wolfram cevherleşmeleri de tespit edilmiştir. Bursa ilinde önemli bakır-kurşun çinko sahaları yer almaktadır. Bakır-kurşun-çinko cevherleşmeleri yoğun olarak İnegöl ilçesinde gözlenmektedir. Bunlardan en önemlileri İnegöl ilçesindeki Hayriye ve Saadet Köyleri sahalarındaki cevherleşmeleridir. -
Bursa-Orhangazi Yakinlarinda Bir Yapi Kalintisi; Ortaköy Hamami
BURSA-ORHANGAZİ YAKINLARINDA BİR YAPI KALINTISI; ORTAKÖY HAMAMI The Remains of a Building; Ortakoy Bath, in Bursa-Orhangazi District A. Mehmet AVUNDUK n this article, the building remains in the Marmara region will be discussed in Bursa I Orhangazi Ortaköy district. Building on the information is not available so far except for some local resources. This building remains dated to the 14th-15 th centuries as the structure of the early Ottoman bath. An inscription indicating the date of the structure and function is not available or no trace. But in this context, the external appearance in terms of its layout, and decorate with the similarity of many structures that dated to the period in question. The article, details of the building and around the Bursa will be discussed the properties with structures similar to the Early Ottoman period. Yrd. Doç. Dr., Sakarya Üniversitesi, Güzel Sanatlar Fakültesi, Geleneksel Türk El Sanatları Bölümü Öğretim Üyesi 2 BURSA-ORHANGAZİ YAKINLARINDA BİR YAPI KALINTISI; ORTAKÖY HAMAMI akalemize konu olan ve hamam olduğu örnekleri hala hatırlardadır. Bu durumu teyit Mdüşünülen yapı kalıntısı Bursa iline bağlı eden somut izler olarak köyü çepeçevre saran Ortaköy sınırları içinde yer almaktadır. Ortaköy, yıllanmış ağaçların yer aldığı geniş zeytinlikler, Bursa’ya 52 km, Orhangazi ilçesine 7 km köy merkezinde mimarisi ile eski dönemlere ait uzaklıkta olup güneyde Cihanköy, güneydoğuda olduğu hissedilen yenilenmiş mescit, köyün Yeniköy ve kuzeyde Sugören köyleri ile dışındaki Osmanlı mezarlığı, bu mezarlığın çevrilidir. Bu üç köyün tam ortasında bulunduğu yakınındaki yolun kenarında Ermenice yazılı için Ortaköy olarak adlandırılmıştır. Yalova– bazı mezar taşları ve burada ele aldığımız Bursa yolu üzerinde her iki ili ayıran doğal hat hamam kalıntısı gibi göz önündeki örnekler, kabul edilen Samanlı Dağlarının güney köyün geçmiş tarihinin izlerini sürmemize eteklerine kurulmuş olan Ortaköy, köklü bir yardımcı olur. -
GIS Mapping of Biogas Potential from Animal Wastes in Bursa, Turkey
74 February, 2015 Int J Agric & Biol Eng Open Access at http://www.ijabe.org Vol. 8 No.1 GIS mapping of biogas potential from animal wastes in Bursa, Turkey Gokhan Ozsoy1*, Ilknur Alibas2 (1. Department of Soil Science and Plant Nutrition, Faculty of Agriculture, Uludag University, 16059 Bursa, Turkey; 2. Department of Biosystems Engineering, Faculty of Agriculture, Uludag University, 16059 Bursa, Turkey) Abstract: This paper introduces biogas potential of animal waste in Bursa, an important agricultural, industrial and tourism centre in northwest Turkey. This research has focused on Bursa’s biogas potential from animal wastes. The potential quantity of electric energy, the potential amount of biogas as well as potential bio-electric energy per capita to be obtained from animal wastes were studied. If the evaluation of biogas potential is conducted thoroughly, 1.12% of the electricity consumption of Bursa can be met with the conversion of biogas from animal wastes into electricity. This study also revealed that the power for 95% of street lighting, approximately twofold of the electricity consumed in official apartments and all of the agricultural irrigation operations can be provided with electrical energy obtained from biogas obtained from animal wastes in Bursa. In addition, the research efficiency was improved by creating thematic maps in GIS, which enabled differences in data among the districts to be observed more clearly. Keywords: animal waste, biogas, energy, Bursa, GIS mapping DOI: 10.3965/j.ijabe.20150801.010 Citation: Ozsoy G, Alibas I. GIS mapping of biogas potential from animal wastes in Bursa, Turkey. Int J Agric & Biol Eng, 2015; 8(1): 74-83. -
Data Collection Survey for Disaster Resilient Urban Planning in Turkey
DATA COLLECTION SURVEY FOR DISASTER RESILIENT URBAN PLANNING IN TURKEY FINAL REPORT (SUMMARY) MAY 2014 JAPAN INTERNATIONAL COOPERATION AGENCY ORIENTAL CONSULTANTS CO., LTD. NIKKEN SEKKEI LTD. 7R INTERNATIONAL TOTAL ENGINEERING CORPORATION CR(3) 14-018 DATA COLLECTION SURVEY FOR DISASTER RESILIENT URBAN PLANNING IN TURKEY FINAL REPORT (SUMMARY) MAY 2014 JAPAN INTERNATIONAL COOPERATION AGENCY ORIENTAL CONSULTANTS CO., LTD. NIKKEN SEKKEI LTD. INTERNATIONAL TOTAL ENGINEERING CORPORATION Table of Contents Location Map Abbreviations 1. SURVEY BACKGROUND ................................................................................................................ 1-1 1.1. SURVEY BACKGROUND ............................................................................................................................... 1-1 1.2. SURVEY OBJECTIVES .................................................................................................................................. 1-1 1.3. SURVEY SCHEDULE .................................................................................................................................... 1-2 1.4. IMPLEMENTING AGENCIES .......................................................................................................................... 1-2 2. CURRENT STATE OF THE DISASTER PREVENTION SECTOR IN TURKEY ........................................... 2-1 2.1. AFAD .................................................................................................................................................... -
An Ottoman Global Moment
AN OTTOMAN GLOBAL MOMENT: WAR OF SECOND COALITION IN THE LEVANT A Dissertation submitted to the Faculty of the Graduate School of Arts and Sciences of Georgetown University in partial fulfillment of the requirements for the Degree of Doctor of Philosophy In History By Kahraman Sakul, M.A Washington, DC November, 18, 2009 Copyright 2009 by Kahraman Sakul All Rights Reserved ii AN OTTOMAN GLOBAL MOMENT: WAR OF SECOND COALITION IN THE LEVANT Kahraman Sakul, M.A. Dissertation Advisor: Gabor Agoston, Ph.D. ABSTRACT This dissertation aims to place the Ottoman Empire within its proper context in the Napoleonic Age and calls for a recognition of the crucial role of the Sublime Porte in the War of Second Coalition (1798-1802). The Ottoman-Russian joint naval expedition (1798-1800) to the Ionian Islands under the French occupation provides the framework for an examination of the Ottoman willingness to join the European system of alliance in the Napoleonic age which brought the victory against France in the Levant in the War of Second Coalition (1798-1802). Collections of the Ottoman Archives and Topkapı Palace Archives in Istanbul as well as various chronicles and treatises in Turkish supply most of the primary sources for this dissertation. Appendices, charts and maps are provided to make the findings on the expedition, finance and logistics more readable. The body of the dissertation is divided into nine chapters discussing in order the global setting and domestic situation prior to the forming of the second coalition, the Adriatic expedition, its financial and logistical aspects with the ensuing socio-economic problems in the Morea, the Sublime Porte’s relations with its protectorate – The Republic of Seven United Islands, and finally the post-war diplomacy. -
Bulletin of the Mineral Research and Exploration
Bulletin of MTA (2013) 147: 1-17 Bulletin of the Mineral Research and Exploration http://bulletin.mta.gov.tr THE SEGMENT STRUCTURE OF SOUTHERN BRANCH OF THE NORTH ANATOLIAN FAULT AND PALEOSEISMOLOGICAL BEHAVIOUR OF THE GEMLİK FAULT, NW ANATOLIA Selim ÖZALPa,*, Ömer EMREb and Ahmet DOĞANc a General Directorate of Mineral Research and Exploration, Dept. of Geological Researches, 06800, Ankara, Turkey b Fugro-Sial Geoscience Consulting and Engineering Ltd Co., Farabi Sk., No: 40/4, Çankaya, Ankara, Turkey c General Directorate of Combating to Desertification and Erosion Control, Ankara, Turkey ABSTRACT Keywords: The North Anatolian Fault (NAF), which is an intra-continental transform fault, is divided North Anatolian into two branches as Northern and Southern branches in Marmara Region. The southern Fault-Southern Branch, Gemlik Fault, branch which separates from each other by rightward stepovers between Bandirma and paleoseismology. Dokurcun valley is formed by three main fault segments as Geyve, İznik and Gemlik from East to West. The length of fault segments ranges between 40 and 57 km and GPS data in Southern branch propose a 5 mm/year slip rate. Two surface faulting events were observed during paleoseismological excavations which had been carried out on 40 km long Gemlik segment and these can be correlated with earthquakes that occurred in 1857 and 1419. The recurrence interval between the last two earthquakes in Gemlik Fault is 438 years. Findings indicate that Gemlik fault was also included into surface faulting of the earthquake in 1419 which its presence is known in İznik fault. At least 95 km long multi-segment surface faulting developed in this earthquake. -
Dahlia Greidinger Symposium
Dahlia Greidinger International Symposium 2009 1 The Dahlia Greidinger International Symposium - 2009 Crop Production in the 21st Century: Global Climate Change, Environmental Risks and Water Scarcity March 2-5, 2009 Technion-IIT, Haifa, Israel Symposium Proceedings Editors: A. Shaviv, D. Broday, S. Cohen, A. Furman, and R. Kanwar Technical Editor: Lee Cornfield Organized and Supported by: BARD, The United States - Israel Binational Agricultural Research and Development Fund Workshop No. W-79-08 and The Dahlia Greidinger Memorial Fund 2 Crop Production in the 21st Century The Dahlia Greidinger International Symposium - 2009 Crop Production in the 21st Century: Global Climate Change, Environmental Risks and Water Scarcity Regional Organizing Committee Chair- Prof. A. Shaviv, Technion-IIT Prof. Emeritus J. Hagin, Technion-IIT Dr. D. Broday, Technion-IIT Dr. S. Cohen, ARO, Volcani Center Dr. A. Furman, Technion-IIT Mr. G. Kalyan, Fertilizers & Chem. Ltd., Haifa Dr. A. Aliewi, House of Water and Environment, Ramallah Mrs. Lee Cornfield, Technion– IIT – Symposium secretary Scientific Committee In addition to the scientists listed above, the scientific committee included: Co-Chair Prof. R. Kanwar, Iowa State University Prof. R. Mohtar, Purdue University Prof. M. Walter, Cornell University Dahlia Greidinger International Symposium 2009 3 Preface The symposium aimed to re-examine knowledge gaps and R&D directions and needs, and to identify –based on this examination– possible modes and new approaches for coping with the increasing severity of water scarcity and quality and soil degradation. These issues were considered in terms of their effects on food security, that is, the foreseen difficulties in Crop and Food Production in a World of Global Changes, Environmental Problems, and Water Scarcity.