Herschel/Planck Consolidated Report on Mission Analysis Issue 3.1 PT-MA-RP-0010-OPS-GMA
Total Page:16
File Type:pdf, Size:1020Kb
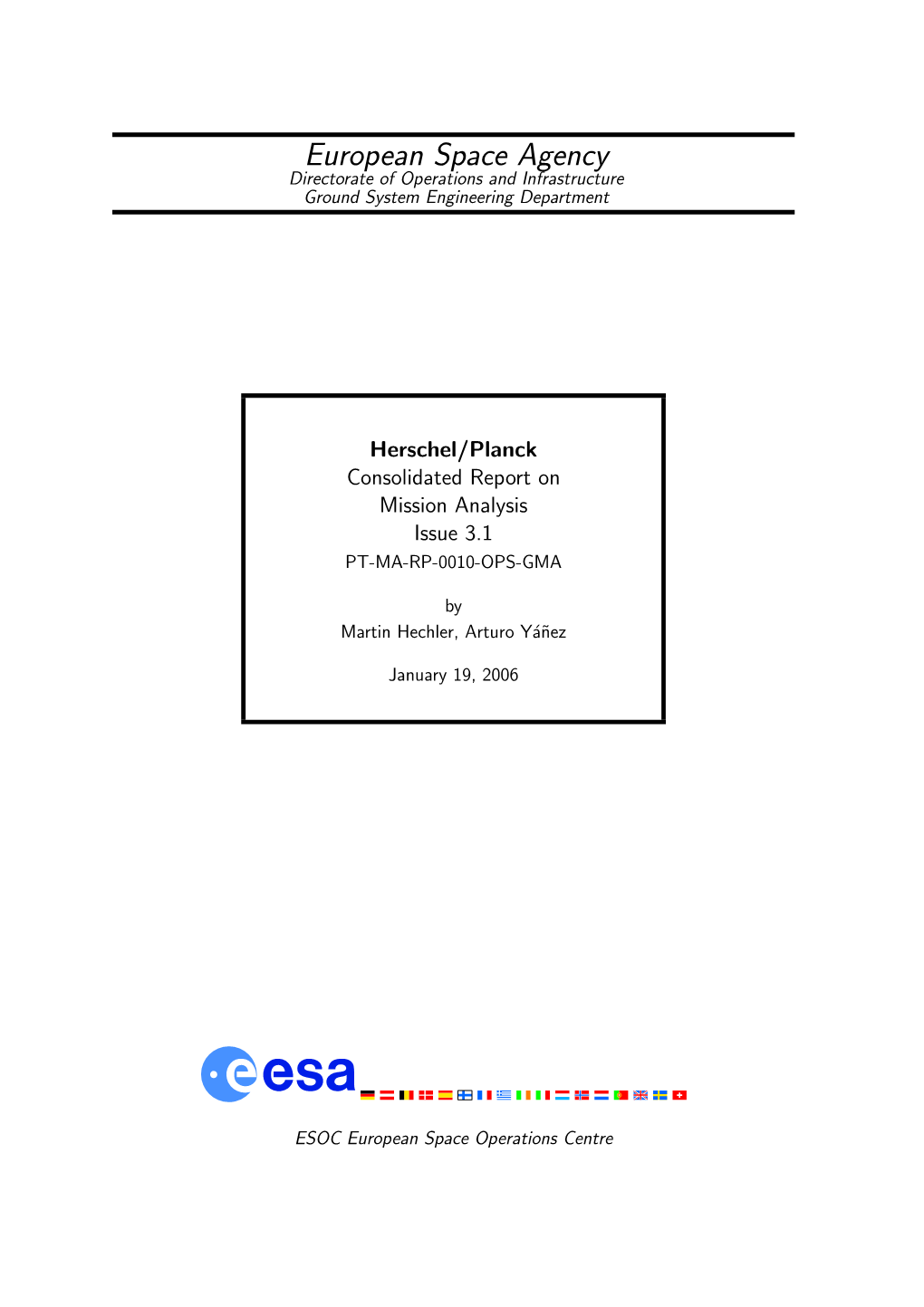
Load more
Recommended publications
-
A European Cooperation Programme
Year 32 • Issue #363 • July/August 2019 2,10 € ESPAÑOLA DE InternationalFirst Edition Defence of the REVISTA DEFEandNS SecurityFEINDEF ExhibitionA Future air combat system A EUROPEAN COOPERATION PROGRAMME BALTOPS 2019 Spain takes part with three vessels and a landing force in NATO’s biggest annual manoeuvres in the Baltic Sea ESPAÑOLA REVISTA DE DEFENSA We talk about defense NOW ALSO IN ENGLISH MANCHETA-INGLÉS-353 16/7/19 08:33 Página 1 CONTENTS Managing Editor: Yolanda Rodríguez Vidales. Editor in Chief: Víctor Hernández Martínez. Heads of section. Internacional: Rosa Ruiz Fernández. Director de Arte: Rafael Navarro. Parlamento y Opinión: Santiago Fernández del Vado. Cultura: Esther P. Martínez. Fotografía: Pepe Díaz. Sections. Nacional: Elena Tarilonte. Fuerzas Armadas: José Luis Expósito Montero. Fotografía y Archivo: Hélène Gicquel Pasquier. Maque- tación: Eduardo Fernández Salvador. Collaborators: Juan Pons. Fotografías: Air- bus, Armada, Dassault Aviation, Joaquín Garat, Iñaki Gómez, Latvian Army, Latvian Ministry of Defence, NASA, Ricardo Pérez, INDUSTRY AND TECHNOLOGY Jesús de los Reyes y US Navy. Translators: Grainne Mary Gahan, Manuel Gómez Pumares, María Sarandeses Fernández-Santa Eulalia y NGWS, Fuensanta Zaballa Gómez. a European cooperation project Germany, France and Spain join together to build the future 6 fighter aircraft. Published by: Ministerio de Defensa. Editing: C/ San Nicolás, 11. 28013 MADRID. Phone Numbers: 91 516 04 31/19 (dirección), 91 516 04 17/91 516 04 21 (redacción). Fax: 91 516 04 18. Correo electrónico:[email protected] def.es. Website: www.defensa.gob.es. Admi- ARMED FORCES nistration, distribution and subscriptions: Subdirección General de Publicaciones y 16 High-readiness Patrimonio Cultural: C/ Camino de Ingenieros, 6. -
ISDEFE-Annual Report 2015.Pdf
ANNUAL REPORT ANNUAL REPORT 2015 This document is the 2015 Isdefe Annual Report on the corporate governance of the company and its performance over the course of the year in three main areas: financial, social and environmental. Isdefe publishes a corporate social responsibility (CSR) report annually based on the directives and principles of the Global Reporting Initiative (GRI), which specifies the methods for determining the content, scope and coverage of the report and ensures the quality of the information presented. In keeping with its environmental commitment, the paper versions of the 2015 Annual Report and the Executive Summary are issued in very limited quantities. The digital formats of both documents are available on our website, www.isdefe.es. The information contained in this document complies with the G4 “Comprehensive” requirements specified by the GRI. Business Development Directorate Tel.: 91 2711140 Corporate Social Responsibility Unit Fax: 91 4114703 Beatriz de Bobadilla, 3. 28040 Madrid Website: www.Isdefe.es Tax id number: A78085719 E-mail: [email protected] CONTENTS LETTER FROM THE LETTER FROM 05 PRESIDENT 07 THE CEO ACTIVITIES THE ORGANIZATION 09 25 REPORT COMMITMENT TO CORPORATE 73 OUR STAKEHOLDERS 105 GOVERNANCE ANNUAL ABOUT THIS 119 ACCOUNTS 125 REPORT LETTER FROM THE PRESIDENT I am pleased to present the 2015 Isdefe Annual Corporate Social Responsibility Report, which reflects Management’s efforts to achieve its sustainability objectives. Isdefe, which in 2015 marked the 30th anniversary of its founding in 1985, has evolved positively to become the benchmark in-house public sector provider of outstanding technology and strategic consulting services in every area where it conducts its business, but particularly in the Defence and Security industries, from which Isdefe has been able to expand its know-how, synergies and experience to other areas of government. -
ESA's New Cebreros Station Ready to Support Venus Express
ESAE ’s New Cebre ere oso S b r N s A r e w C StationS Ready to t a t i o n R e a d y t SupporS t Venuse u nV p u p s o r Express Cebreros Station Manfred Warhaut, Rolf Martin & Valeriano Claros ESA Directorate of Operations and Infrastructure, ESOC, Darmstadt, Germany SA’s new deep-space radio antenna at Cebreros (near Avila) in Spain was Eofficially inaugurated on 28 September. The new 35 metre antenna is the Agency’s second facility devoted to communications with spacecraft on interplanetary missions or in very distant orbits; the first is at New Norcia in Western Australia. Cebreros’s first task is the tracking of ESA’s Venus Express spacecraft, launched on 9 November. Introduction The construction of ESA's deep-space antenna at Cebreros was completed in record time. The site-selection process began in April 2002, the procurement activities began in February 2003, and the building work began in Spring 2004 on the site of a former NASA ground station. After successful assembly of the antenna structure in November 2004 and the almost flawless acceptance testing of the various infrastructure elements and the radio- frequency components, the new antenna was completed in August 2005, which provided just sufficient time for final testing before being used for the first time to support Venus Express. esa bulletin 124 - november 2005 39 Infrastructure Technical Specifications of the Cebreros Antenna REFLECTOR DISH Diameter: 35 metres Depth: 8 metres Surface contour: shaped parabola Number of panels: 304 on 7 rings Surface accuracy: 0.3 mm rms Weight: 100 tons ANTENNA PEDESTAL Height: 40 metres Weight movable part: 500 tons Total weight: 620 tons OPERATING ENVIRONMENT The novel Cebreros antenna feed concept Temperature: -20°C to + 50°C Relative humidity: 0 – 100% including condensation Wind: up to 50 km/h constant, gusting to 70 km/h Rain: up to 35 mm/h Solar heat: up to 1200 W/m2 MECHANICAL PERFORMANCE Slew range: Azimuth 0 to 540 deg Elevation 0 to 90 deg Slew rate: Both axes 1.0 deg/s max. -
ESTRACK Facilities Manual (EFM) Issue 1 Revision 1 - 19/09/2008 S DOPS-ESTR-OPS-MAN-1001-OPS-ONN 2Page Ii of Ii
fDOCUMENT document title/ titre du document ESA TRACKING STATIONS (ESTRACK) FACILITIES MANUAL (EFM) prepared by/préparé par Peter Müller reference/réference DOPS-ESTR-OPS-MAN-1001-OPS-ONN issue/édition 1 revision/révision 1 date of issue/date d’édition 19/09/2008 status/état Approved/Applicable Document type/type de document SSM Distribution/distribution see next page a ESOC DOPS-ESTR-OPS-MAN-1001- OPS-ONN EFM Issue 1 Rev 1 European Space Operations Centre - Robert-Bosch-Strasse 5, 64293 Darmstadt - Germany Final 2008-09-19.doc Tel. (49) 615190-0 - Fax (49) 615190 495 www.esa.int ESTRACK Facilities Manual (EFM) issue 1 revision 1 - 19/09/2008 s DOPS-ESTR-OPS-MAN-1001-OPS-ONN 2page ii of ii Distribution/distribution D/EOP D/EUI D/HME D/LAU D/SCI EOP-B EUI-A HME-A LAU-P SCI-A EOP-C EUI-AC HME-AA LAU-PA SCI-AI EOP-E EUI-AH HME-AT LAU-PV SCI-AM EOP-S EUI-C HME-AM LAU-PQ SCI-AP EOP-SC EUI-N HME-AP LAU-PT SCI-AT EOP-SE EUI-NA HME-AS LAU-E SCI-C EOP-SM EUI-NC HME-G LAU-EK SCI-CA EOP-SF EUI-NE HME-GA LAU-ER SCI-CC EOP-SA EUI-NG HME-GP LAU-EY SCI-CI EOP-P EUI-P HME-GO LAU-S SCI-CM EOP-PM EUI-S HME-GS LAU-SF SCI-CS EOP-PI EUI-SI HME-H LAU-SN SCI-M EOP-PE EUI-T HME-HS LAU-SP SCI-MM EOP-PA EUI-TA HME-HF LAU-CO SCI-MR EOP-PC EUI-TC HME-HT SCI-S EOP-PG EUI-TL HME-HP SCI-SA EOP-PL EUI-TM HME-HM SCI-SM EOP-PR EUI-TP HME-M SCI-SD EOP-PS EUI-TS HME-MA SCI-SO EOP-PT EUI-TT HME-MP SCI-P EOP-PW EUI-W HME-ME SCI-PB EOP-PY HME-MC SCI-PD EOP-G HME-MF SCI-PE EOP-GC HME-MS SCI-PJ EOP-GM HME-MH SCI-PL EOP-GS HME-E SCI-PN EOP-GF HME-I SCI-PP EOP-GU HME-CO SCI-PR -
Cuadernos De Estrategia 170. El Sector Espacial En España
Cuadernos de Estrategia 170 Instituto Español El sector espacial en España. de Estudios Evolución y perspectivas Estratégicos MINISTERIO DE DEFENSA Cuadernos de Estrategia 170 Instituto Español El sector espacial en España. de Estudios Evolución y perspectivas Estratégicos MINISTERIO DE DEFENSA CATÁLOGO GENERAL DE PUBLICACIONES OFICIALES http://publicacionesoficiales.boe.es/ Edita: SECRETARÍA GENERAL TÉCNICA http://publicaciones.defensa.gob.es/ © Autor y editor, 2014 NIPO: 083-14-236-5 (edición papel) NIPO: 083-14-235-X (edición libro-e) ISBN: 978-84-9091-006-1 (edición papel) ISBN: 978-84-9091-005-4 (edición libro-e) Depósito Legal: M-30595-2014 Fecha de edición: diciembre 2014 Imprime: Imprenta del Ministerio de Defensa Las opiniones emitidas en esta publicación son exclusiva responsabilidad de los autores de la misma. Los derechos de explotación de esta obra están amparados por la Ley de Propiedad Intelectual. Ninguna de las partes de la misma puede ser reproducida, almacenada ni transmitida en ninguna forma ni por medio alguno, electrónico, mecánico o de grabación, incluido fotocopias, o por cual- quier otra forma, sin permiso previo, expreso y por escrito de los titulares del © Copyright. En esta edición se ha utilizado papel 100% reciclado libre de cloro. ÍNDICE Página Introducción Vicente Gómez Domínguez Sinopsis ....................................................................................................................... 15 Resumen de los capítulos ...................................................................................... -
Optoelectronic COTS Technologies for Planetary Exploration
Optoelectronic COTS Technologies for Planetary exploration Héctor GUERRERO [email protected] INTA - Instituto Nacional de Técnica Aeroespacial SPAIN EUROPLANET Meeting (FMI / Helsinki) – May 26th, 2011 Mission SpanishSpanish PublicPublic ResearchResearch OrganizationOrganization specializedspecialized inin aerospaceaerospacetechnical technical researchresearch andand developmentdevelopment INTA’s Facilities CebrerosCebreros (Avila)(Avila) •• INTA/ESA INTA/ESA RobledoRobledo dede ChavelaChavela (Madrid)(Madrid) •• INTA/NASA INTA/NASA 2,5 km VillafrancaVillafranca (Madrid)(Madrid) INTA •• ESACESAC ((EuropeanEuropean SpaceSpace AstronomyAstronomy CentreCentre)) Torrejón de Ardoz (Madrid) GranadaGranada (Airport)(Airport) •• Flight Flight testingtesting SevillaSevilla “El“El Arenosillo”Arenosillo” (Huelva)(Huelva) •• CEDEA CEDEA MaspalomasMaspalomas (Gran(Gran Canaria)Canaria) Space Technology Related Activities Participation of INTA in MARS Exploration Next ‘Martian’ opportunities for INTA Phobos Sample Return 1 Mars Science Laboratory Curiosity 2 MetNet REMS Meteorological Station (+ FMI/Finland) Precursor 3 RAMAN Spectrometer Exomars 2016 (P/L for the ‘Soft’ Lander) + 2018 (Rover) Optoelectronic COTS Technologies for Planetary exploration EUROPLANET Meeting (FMI / Helsinki), May 26th 2011 OUTLINE 1. Why optoelectronic COTS for Space Exploration? 2. OWLS: A driven force for Optoelectronics 3. Irradiation of Optoelectronic components 4. A pragmatic strategy: Systems and Sensors 5. Developing of Radiation Monitors 6. Conclusions Optoelectronic -
Analiza Sektora Kosmicznego Wybranych Państw
Analiza sektora kosmicznego wybranych państw Warszawa, 14.05.2021 Autorzy tekstów: Marta Balcer, dr Aleksandra Bukała, Zbigniew Burdzy, John Hall, Patrycja Karwowska, dr inż. Jakub Kowalewski, Anna Stańczyk, Marzena Świderska, Oskar Zdunek Departamet Strategii i Współpracy Międzynarodowej Polska Agencja Kosmiczna, 2021 2 Spis treści Wprowadzenie 5 Austria 6 Belgia 16 Czechy 24 Francja 32 Hiszpania 42 Niemcy 52 Słowacja 64 Ukraina 70 USA 78 Węgry 82 Wielka Brytania 94 Włochy 102 Budżety wybranych państw 110 Słownik najważniejszych skrótów 112 Źródła 114 3 4 Wprowadzenie Celem niniejszego opracowania jest przybliżenie informacji na temat rynku kosmicznego w wybranych państwach. Raport zawiera analizę sektora kosmicznego dwunastu krajów – Austrii, Belgii, Czech, Francji, Hiszpanii, Niemiec, Słowacji, Ukrainy, USA, Węgier, Wielkiej Brytanii i Włoch. Raport stanowi kolejną część dokumentu „ANALIZA SEKTORA KOSMICZNEGO WYBRANYCH KRAJÓW EUROPY ŚRODKOWO- WSCHODNIEJ”, opracowanego przez Departament Strategii i Współpracy Międzynarodowej POLSA w 2018 r. Rozszerzono i zaktualizowano dane dotyczące sektora kosmicznego Austrii, Belgii, Czech, Niemiec, Słowacji, Wielkiej Brytani i Węgier. Dodatkowo dokonano analizy rynków kosmicznych Francji, Hiszpanii i Włoch – trzech z pięciu głównych graczy rynku kosmicznego w Europie. Ponadto publikacja została uzupełniona o rozdział charakteryzujący rynek Stanów Zjednoczonych Ameryki Północnej-globalnego lidera w kwestii eksploracji przestrzeni kosmicznej. Każdy rozdział opisuje sektor kosmiczny jednego z państw -
Esa Annual Report 2006 Facilities 93 Annrep06 Facilities 7/25/07 3:49 PM Page 94
AnnRep06_Facilities 7/25/07 3:49 PM Page 93 Facilities esa Annual Report 2006 Facilities 93 AnnRep06_Facilities 7/25/07 3:49 PM Page 94 ESTEC ESTEC was extremely busy, with the simultaneous system and verification testing of several ESA spacecraft. In addition to the newly arrived Herschel Payload Module structural model, testing of the Automated Transfer Vehicle (ATV), the Herschel structural model and the Planck telescope continued, resulting in a very high workload for the Centre. In view of the ATV’s 2007 launch, astronaut training was also performed for late access to space-vehicle parts. ESTEC Test Centre A380 luggage pallet using the Hydra (hydraulic shaker) in July. The novel aspect of this test was the simulta- Elements of the Vega small launcher were also tested. neous introduction in six degrees of freedom of The payload mechanical dummy and the upper- transient test excitations that have been measured composite mechanical model underwent vibration during flight, for verification of the loaded pallet. The testing from June to August, and the latter, including the use of this type of aeronautical testing for the verifica- fairing, underwent acoustic tests in the Large European tion of satellite hardware will now be considered. Acoustic Facility (LEAF) in October. In parallel with the spacecraft and subsystem testing, the Engineering Laboratories continuous replacement and updating of ageing test equipment continued with the replacement of the mass- Power Laboratory property measurement facility, acquired from APCO Several ideas and concepts developed within the power- Switzerland, which came into operation at the end of systems and power-conditioning laboratories at ESTEC February. -
SPACE and SECURITY POLICY in EUROPE IAI Research
Istituto Affari Internazionali SSppaaccee aanndd SSeeccuurriittyy PPoolliiccyy iinn EEuurrooppee ROME, NOVEMBER 2003 RESEARCH TEAM Istituto Affari Internazionali (IAI), Roma, coordinator European Union Institute for Security Studies (EU-ISS), Paris Centre for European Reform (CER), London Deutsche Gesellschaft für Auswärtige Politik (DGAP), Berlin Fondation pour la Recherche Stratégique (FRS), Paris Institut d’Etudes Européennes (IEE) of the University of Louvain RESEARCH GROUP Klaus Becher (EU-ISS, Paris) Jean-Pierre Darnis (IAI, Rome) Giovanni Gasparini (IAI, Rome) Daniel Keohane (CER, London) Michel Liégeois (ULV, Institut d’Etudes Européennes, CECRI, Louvain) Michele Nones (IAI, Rome) Xavier Pasco (FRS, Paris) Niklas Reinke (DGAP, Berlin) Henning Riecke (DGAP, Berlin) Burkard Schmitt (EU-ISS, Paris) Stefano Silvestri (IAI, Rome) Isabelle Sourbes-Verger (FRS-CNRS, Paris) Jost Vielhaber (DGAP, Berlin) CONTRIBUTIONS Angela Garrigos de la Uz Mike Winnerstig (FOI, Stockholm) A Study funded by the European Space Agency (ESA) Esa Technical Officier: Simonetta Cheli SPACE AND SECURITY POLICY IN EUROPE IAI Research Index Introduction.................................................................................................................................. 7 1 For a new concept of space security in Europe.............................................................................11 A dual approach : National defence space assets, European civilian space policy..............................12 1.2 A broader concept of space security. Internal -
Improved Spacecraft Tracking and Navigation Using a Portable Radio
Improved Spacecraft Tracking and Navigation Using a Portable Radio Science Receiver Melissa Soriano, Christopher Jacobs, Juan Lobo Garcia, Cristina García-Miró Robert Navarro, Charles Naudet, INTA/NASA Stephen Rogstad, Leslie White, Madrid Deep Space Communications Complex Susan Finley, Charles Goodhart, Madrid, Spain Elliott Sigman, Joseph Trinh Jet Propulsion Laboratory Mattia Mercolino, Roberto Maddè California Institute of Technology European Space Agency Pasadena, CA 91109 European Space Operations Center 818-393-7632 Darmstadt, Germany [email protected] Abstract— The Portable Radio Science Receiver (PRSR) is a 1. INTRODUCTION suitcase-sized open-loop digital receiver designed to be small and easy to transport so that it can be deployed quickly and Interplanetary navigation uses four primary types of easily anywhere in the world. The PRSR digitizes, down- measurements for orbit determination: Range, Doppler, converts, and filters using custom hardware, firmware, and Delta Differential One-way Range, and onboard optical. software. Up to 16 channels can be independently configured Range is a measurement of the distance to the spacecraft and recorded with a total data rate of up to 256 Mbps. The and is obtained using the difference in time between when a design and implementation of the system's hardware, signal is sent and received. Doppler is a measurement of the firmware, and software is described. To minimize costs and time to deployment, our design leveraged elements of the radial velocity of the spacecraft using the Doppler shift of hardware, firmware, and software designs from the existing the signal [1]. Delta Differential One-Way (DOR) range is full-sized operational (non-portable) Radio Science Receivers a measurement of the angular position and velocity of the (RSR) and Wideband VLBI Science Receivers (WVSR), which spacecraft using station-differenced one-way range [2]. -
SATELLITE SEOSAT Ingenio
No. 45 I MAY I 2020 SATELLITE SEOSAT ingenio LATEST NEWS OPINION SOLAR ORBITER GLORIA LASO ON ITS WAY TO THE SUN REMOTE TEACHING, A CHALLENGE FOR TEACHERS AND STUDENTS THE DARK DUNES OF THE MOREUX CRATER The Moreux crater on Mars demonstrates many and disorderly, the result of its erosion over the intriguing geological processes and features. It sits period of Martian history. Its edge, in the form of at the northern extreme of the Terra Sabaea, a large an egg, is broken, its dark walls are furrowed, wavy area of the Red Planet that is splattered with impact and mottled. In its centre is a prominent group of craters and covered with glacial flows, dunes, rough ‘peaks’ created as material from the floor of the ground and networks of intricate crests. Compared crater rebounds and rises upwards following the with other impact craters, both on Mars and on initial impact. Earth, the Moreux crater seems a bit deformed Text: ESA. Photo: NASA, ESA. IberEspacio Tecnología Aeroespacial A booming market: pairing opportunities for small satellites and small launchers THE INTERNATIONAL SPACE SECTOR has changed substantially in the last 20 years. The current trend known as New Space emerged at the start of the century with the appearance of Space companies (mainly founded by millionaires) with the support of NASA and Venture Capital funds that enabled new commercial opportunities to open up. Among examples of this trend are SpaceX, Blue Origin, Virgin Galactic and Planetlab. What prevailed was a “traditional” development with some different approaches and the entry of private money in contrast to the usual organic growth in the sector. -
Venus Express CRMA Issue 1, Rev 0
a directorate of technical and operational support ground systems engineering department mission support office mission analysis office VEX-ESC-RP-5500 Venus Express: Consolidated Report On Mission Analysis (Issue 3) by J. M. Sánchez Pérez J. Rodríguez Canabal April, 2005 European Space Operations Centre Robert-Bosch-Str. 5 D - 64293 Darmstadt Doc. Title: Venus Express: Consolidated Report On Mission Analysis Issue: 3 Doc Ref.: VEX-ESC-RP-5500 Rev.: 0 Date: April, 2005 Page: ii Venus Express: Consolidated Report On Mission Analysis Issue 3, Rev.0, 2005 ESA/ESOC, 64293 Darmstadt, Germany, April, 2005 European Space Agency VEX-ESC-RP-5500 Agence spatiale européenne ESOC Mission Analysis Office Robert-Bosch-Strasse 5, 64293 Darmstadt, Germany ESA-OPS-GA Tel. +49-6151-90-2059; Fax +49-6151-90-2625 Doc. Title: Venus Express: Consolidated Report On Mission Analysis Issue: 3 Doc Ref.: VEX-ESC-RP-5500 Rev.: 0 Date: April, 2005 Page: iii Document Approval Prepared by Address Code Signature Date J.Rodríguez Canabal ESA/OPS/GA 05.04.2005 J.M. Sánchez Pérez ESA/OPS/GA 05.04.2005 Approved by Address Code Signature Date J.Rodríguez Canabal ESA/OPS/GA 05.04.2005 European Space Agency VEX-ESC-RP-5500 Agence spatiale européenne ESOC Mission Analysis Office Robert-Bosch-Strasse 5, 64293 Darmstadt, Germany ESA-OPS-GA Tel. +49-6151-90-2059; Fax +49-6151-90-2625 Doc. Title: Venus Express: Consolidated Report On Mission Analysis Issue: 3 Doc Ref.: VEX-ESC-RP-5500 Rev.: 0 Date: April, 2005 Page: iv Change Record Date Issue Rev. Page/Para. Description Approval affected authority Update of references R6 and R8.