Hydrosulfide Complexes of the Transition Elements: Diverse Roles in Bioinorganic, Cluster, Coordination, and Organometallic Chemistry
Total Page:16
File Type:pdf, Size:1020Kb
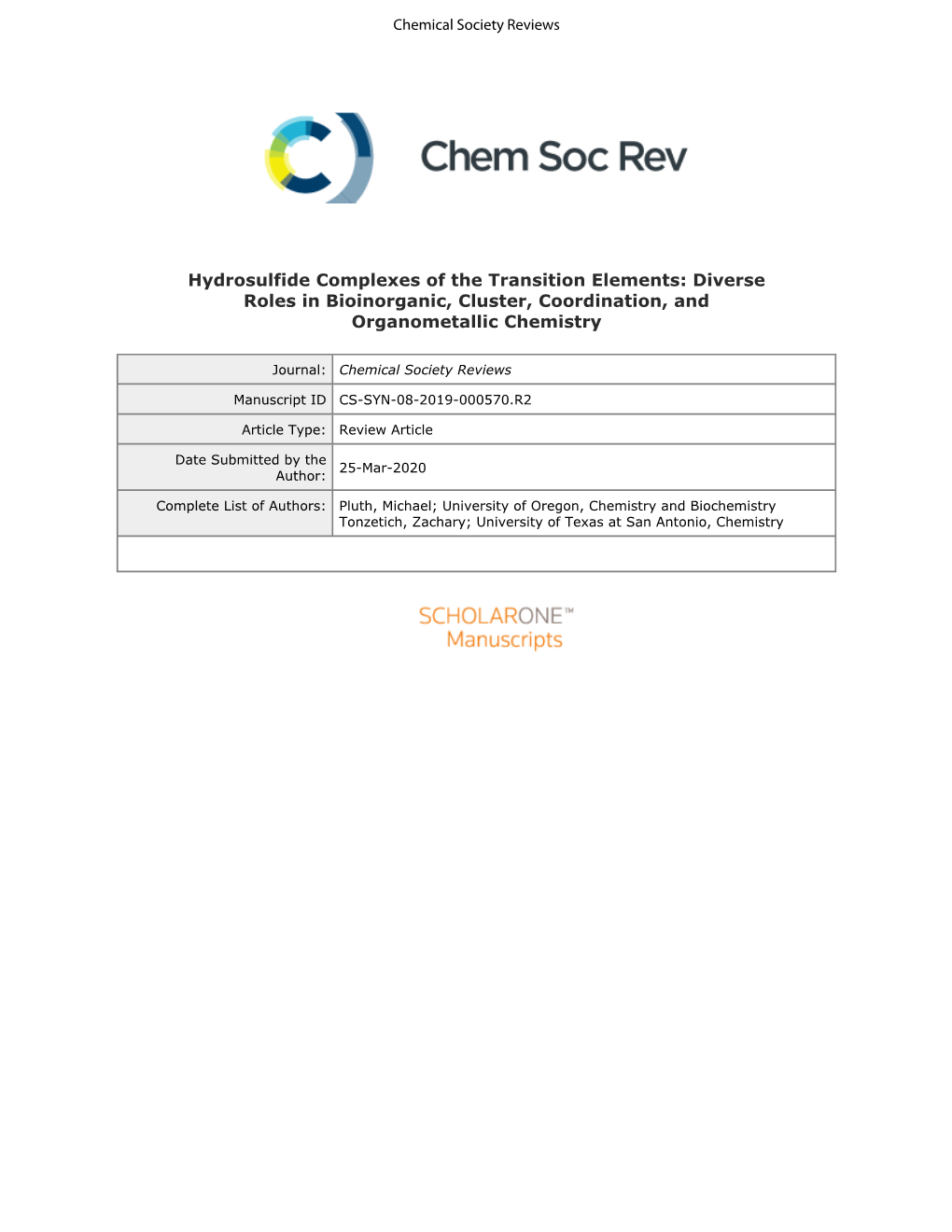
Load more
Recommended publications
-
Chapter 1. Periodic Properties and Variation of Properties
Chapter 1. Periodic Properties and Variation of Properties PAGE NO : 16 Solution 1: Modern periodic law states that the physical and chemical properties of elements are a periodic function of their atomic numbers i.e., if the elements are arranged in the order of their atomic numbers, the elements with similar properties are repeated after definite regular intervals. Concept Insight: The elements are characterized by their atomic number as well as atomic weight. Modern periodic law uses atomic number which is number of protons or number of electrons present in an atom of an element. Solution 2: Modern periodic table consists of eighteen groups and seven periods. Concept Insight: Classification of elements on the basis of increasing atomic number is known as Modern Periodic Table. The vertical columns are called groups and the horizontal rows are called periods. Solution 3: The recurrence of similar properties of elements after certain regular intervals when they are arranged in the order of increasing atomic numbers is called periodicity. Concept Insight: Periodicity in properties is due to the repetition of similar outer electronic configuration of elements at certain regular intervals. Solution 4: In general, the elements belonging to a group have the same number of valence electrons .For example, all the group 1 elements have valency one since they have only one electron in their outermost shell. In general, the elements belonging to a period do not have same valency but their valence shell remains the same. For example, second period has 8 elements with atomic number 3 to 10 but in all of them the valence electrons are present in shell number two. -
Combined Science Chemistry Booklet
COMBINED SCIENCE CHEMISTRY BOOKLET TASK: Complete the questions that your teacher sets on Show My Homework and use the mark scheme at the end of the document to check your responses. Topic 1 – Atomic Structure and the Periodic Table Q1. The periodic table on the Data Sheet may help you to answer these questions. (a) Part of the periodic table is shown below. The letters are not the symbols of the elements. Choose your answers only from the letters shown in the periodic table above. Which letter, A, B, C, D, E or F, represents (i) aluminium (1) Page 1 of 66 (ii) a Group 5 element (1) (iii) an alkali metal (1) (iv) the element with atomic (proton) number of 47 (1) (v) an element with seven electrons in its outer shell? (1) (b) The table shows the boiling points of the Group 7 elements. The elements are arranged in alphabetical order. Group 7 element Name Symbol Boiling point in °C Astatine At 337 Bromine 58 Chlorine Cl -34 Fluorine F -188 Iodine I 184 (i) The symbol for bromine is missing from the table. What is the symbol for bromine? Symbol = _______________ (1) (ii) Arrange these elements in order of decreasing boiling point. The first one and the last one have been done for you. At ______ ______ ______ F Highest boiling Lowest boiling point point Page 2 of 66 (1) (c) The table shows some statements about Group 7 elements. Tick ( ) the two correct statements. Tick ( ) They are halogens. They are metals. They become less reactive down Group 7. -
The Periodic Table: a Very Short Introduction VERY SHORT INTRODUCTIONS Are for Anyone Wanting a Stimulating and Accessible Way Into a New Subject
The Periodic Table: A Very Short Introduction VERY SHORT INTRODUCTIONS are for anyone wanting a stimulating and accessible way into a new subject. They are written by experts, and have been translated into more than 45 different languages. The series began in 1995, and now covers a wide variety of topics in every discipline. The VSI library currently contains over 600 volumes—a Very Short Introduction to everything from Psychology and Philosophy of Science to American History and Relativity—and continues to grow in every subject area. Very Short Introductions available now: ABOLITIONISM Richard S. Newman ACCOUNTING Christopher Nobes ADAM SMITH Christopher J. Berry ADOLESCENCE Peter K. Smith ADVERTISING Winston Fletcher AFRICAN AMERICAN RELIGION Eddie S. Glaude Jr AFRICAN HISTORY John Parker and Richard Rathbone AFRICAN POLITIC Ian Taylor AFRICAN RELIGIONS Jacob K. Olupona AGEING Nancy A. Pachana AGNOSTICISM Robin Le Poidevin AGRICULTURE Paul Brassley and Richard Soffe ALEXANDER THE GREAT Hugh Bowden ALGEBRA Peter M. Higgins AMERICAN CULTURAL HISTORY Eric Avila AMERICAN FOREIGN RELATIONS Andrew Preston AMERICAN HISTORY Paul S. Boyer AMERICAN IMMIGRATION David A. Gerber AMERICAN LEGAL HISTORY G. Edward White AMERICAN NAVAL HISTORY Craig L. Symonds AMERICAN POLITICAL HISTORY Donald Critchlow AMERICAN POLITICAL PARTIES AND ELECTIONS L. Sandy Maisel AMERICAN POLITICS Richard M. Valelly THE AMERICAN PRESIDENCY Charles O. Jones THE AMERICAN REVOLUTION Robert J. Allison AMERICAN SLAVERY Heather Andrea Williams THE AMERICAN WEST Stephen Aron AMERICAN WOMEN’S HISTORY Susan Ware ANAESTHESIA Aidan O’Donnell ANALYTIC PHILOSOPHY Michael Beaney ANARCHISM Colin Ward ANCIENT ASSYRIA Karen Radner ANCIENT EGYPT Ian Shaw ANCIENT EGYPTIAN ART AND ARCHITECTURE Christina Riggs ANCIENT GREECE Paul Cartledge THE ANCIENT NEAR EAST Amanda H. -
1St Halfchemnotes
Science 10 Chemistry Notes Matter and Classification Purpose of classification: to gain a better understanding and appreciate similarities or differences Matter: Has mass and occupies space. We can divide matter into two categories as follows: Matte Pure Mixtur Heterogeneo Homogeneo Elemen Alloy Solutio Metal Nonmeta Compou Ioni Molecul PURE SUBSTANCES Substances that are the same or consistent throughout. Can be a single element or a combination of elements. Elements: Substance composed of only one kind of atom. 109 on the periodic table. Each has a unique international symbol. Can be combined to make other pure substances. Compound: Combination of two or more elements in specific proportions. Once combined the compound acts as one, with consistent chemical and physical properties. Chemical vs. Physical Properties Chemical - describes the reactivity of a substance. Physical -no new substance formed. Has similar properties in each form. Usually a change in phase. ie. Liquid, solid or gas. Chemical Reactions The process that occurus whena subtance or substances reacts to creat a different substance. - involve the production of new substances - involve the flow of energy (exothermic and endothermic) - involves formation of a gas - involves the formation of a solid in a liquid Periodic Table Elements on the periodic table are classified and arranged according to four basic patterns: 1. Atomic number: the number of protons (positively charged particle) in the nucleus of an element. The number of electrons in an atom 2. Metals vs. non-metals: separated by the staircase line. 3. Groups (or families): vertical columns that have similar properties. 4. Periods: horizontal rows which indicate the number of electron shells an atom has. -
Ultra-Thin High-Efficiency Mid-Infrared Transmissive Huygens Meta-Optics
Ultra-thin high-efficiency mid-infrared transmissive Huygens meta-optics The MIT Faculty has made this article openly available. Please share how this access benefits you. Your story matters. Citation Zhang, Li, et al. “Ultra-Thin High-Efficiency Mid-Infrared Transmissive Huygens Meta-Optics.” Nature Communications, vol. 9, no. 1, Dec. 2018. © 2018 The Authors As Published http://dx.doi.org/10.1038/s41467-018-03831-7 Publisher Nature Publishing Group Version Final published version Citable link http://hdl.handle.net/1721.1/118858 Terms of Use Creative Commons Attribution 4.0 International License Detailed Terms http://creativecommons.org/licenses/by/4.0/ ARTICLE Corrected: Author correction DOI: 10.1038/s41467-018-03831-7 OPEN Ultra-thin high-efficiency mid-infrared transmissive Huygens meta-optics Li Zhang1,2, Jun Ding 3,4, Hanyu Zheng1,2, Sensong An4, Hongtao Lin 2, Bowen Zheng4, Qingyang Du2, Gufan Yin2, Jerome Michon2, Yifei Zhang2, Zhuoran Fang2, Mikhail Y. Shalaginov2, Longjiang Deng1, Tian Gu2, Hualiang Zhang4 & Juejun Hu2 The mid-infrared (mid-IR) is a strategically important band for numerous applications ranging 1234567890():,; from night vision to biochemical sensing. Here we theoretically analyzed and experimentally realized a Huygens metasurface platform capable of fulfilling a diverse cross-section of optical functions in the mid-IR. The meta-optical elements were constructed using high-index chalcogenide films deposited on fluoride substrates: the choices of wide-band transparent materials allow the design to be scaled across a broad infrared spectrum. Capitalizing on a two-component Huygens’ meta-atom design, the meta-optical devices feature an ultra-thin profile (λ0/8 in thickness) and measured optical efficiencies up to 75% in transmissive mode for linearly polarized light, representing major improvements over state-of-the-art. -
The Periodic Table on the Data Sheet May Help You to Answer This Question
www.tutorzone.co.uk The periodic table on the Data Sheet may help you to answer this question. 1 (a) Newlands and Mendeleev both designed periodic tables in which the elements were put in the order of their relative atomic masses. When the elements are put in this order a few of them are placed incorrectly when compared with a modern periodic table. (i) Give one example of a pair of elements that would be placed incorrectly if they were in the order of their relative atomic masses. ......................................................... and .......................................................... (1) (ii) Explain why placing these two elements in the order of their relative atomic masses would not be correct. ........................................................................................................................... ........................................................................................................................... (1) (b) In the modern periodic table the elements are put in order of their atomic (proton) numbers. Explain how the positions of the elements in the periodic table are linked to the electronic structure of their atoms. ..................................................................................................................................... ..................................................................................................................................... .................................................................................................................................... -
Chemistry Problem Solving Drill
Inorganic Chemistry - Problem Drill 16: The d and f Block Elements Question No. 1 of 10 Instructions: (1) Read the problem statement and answer choices carefully, (2) Work the problems on paper as needed, (3) Pick the answer, and (4) Review the core concept tutorial as needed. The lanthanide series (4f) of chemical elements comprises the fifteen metallic chemical elements with atomic numbers 57 through 71. Which element is not generally classified with the lanthanides? A. La B. Lu C. U Question D. Gd E. Nd A. Incorrect! La is generally included as the first element in the lanthanide series, despite having no electrons in the f- orbital, since the properties are similar to the f-block lanthanide series (6s2, 5d1). B. Incorrect! Lu is the last element in the lanthanide series and has a fully filled f-orbital (5d14f14). C. Correct! U belongs to the actinide (5f) series of elements. Feedback D. Incorrect! Gd has a 4f7 electron configuration. Hence, belongs with the lanthanide series of elements. E. Incorrect! Nd has a 4f4 electron configuration. Hence belongs to the lanthanide series of elements. Correct answer: U Uranium belongs to the 5f-block of elements, known as the actinides. The actinides are classified together since they have similar properties. Solution RapidLearningCenter.com Rapid Learning Inc. All Rights Reserved Question No. 2 of 10 Instructions: (1) Read the problem statement and answer choices carefully, (2) Work the problems on paper as needed, (3) Pick the answer, and (4) Review the core concept tutorial as needed. Titanium is a chemical element with symbol Ti and atomic number 22. -
Year 7 Science Distance Learning Quiz and Learn Booklet Summer 2
Name:Year 7 Science Form:Distance Learning Quiz and Learn Booklet Summer 2 Name : Form : Year 7 Science home study book Summer 2 2020 Guidance Scientists have proven that when we do not use information we know, the pathways in our brain actually decay (breakdown) - this is what happens when we forget something. By engaging with your study (reading and doing quizzes) you are not just learning new information you are actually holding on to the information you have already have! Well done on all the work you have done to this point, you are not letting all your hard school work and study go to waste! ☺ This booklet has contains: - Information for you to read and make notes on - Practice questions and answers for you to complete and self mark - Paper copies of the online quizzes that all other students will be taking. There are a couple of methods to submit your paper quiz • -If you can, drop it off in the post box in the school reception • -Take a picture and email it to your teacher Your responses in this quiz allows your teacher to give you specific instructions on how to improve, only by doing this can we make sure you make the progress you deserve! If you have any questions please email your teacher. All done for this Week Learn Complete? Quiz Complete? week? ☺ 1 (1st June) Reproductive Systems and Puberty Bio Week 1 2 (8th June) Menstrual Cycle and Fertilisation Bio week 2 3 (15th June) Diffusion Bio week 3 4 (22nd June) Atoms, Elements and Compounds Chemistry week 4 5 (29th June) The Periodic Table Chemistry week 5 6 (6th July) Forces Physics week 6 7 (13th July) Moments and Deformation Physics week 7 Answers Adolescence In-text questions A The period of time in which a person changes from a child into an adult. -
C2.2 What Does the Periodic Table Tell Us About
1(a). Joe does some research about Group 1 elements of the Periodic Table. Joe finds out the electron arrangement for the atoms of these elements. Element Electron arrangement lithium 2.1 sodium 2.8.1 potassium 2.8.8.1 Describe the similarities and differences between the electron arrangement in the atoms of these elements. [3] (b). Joe uses the Periodic Table to make a prediction about the order of reactivity of the three elements. Which order of reactivity for the three elements is correct? Put a tick (✔) in the box next to the correct answer. lithium > sodium > potassium lithium < potassium < sodium potassium > sodium > lithium lithium < sodium > potassium [1] © OCR 2019. 1 of 52 PhysicsAndMathsTutor.com 2. Lithium is an element in Group 1 of the Periodic Table. Lithium reacts with fluorine gas to form lithium fluoride. (i) Complete the word and symbol equation for the reaction. [2] (ii) Draw straight lines to join each substance to its correct description. [2] © OCR 2019. 2 of 52 PhysicsAndMathsTutor.com 3(a). Mendeleev organised the elements into the first Periodic Table. The diagram shows some elements from Mendeleev's Groups 2 and 3. Mendeleev left gaps in his table. Two gaps are shown in Group 3. Explain why these gaps were so important. [2] (b). Two of the elements in Mendeleev's Group 2 are not in Group 2 of the modern Periodic Table. Identify the elements and state where they are found in the modern Periodic Table. [2] © OCR 2019. 3 of 52 PhysicsAndMathsTutor.com 4. Len looks up data about the sizes of atoms of chlorine and some other Group 7 elements. -
INTRODUCTORY CHEMISTRY 1 Paul M
Education: Chemistry CHE 01 INTRODUCTORY CHEMISTRY 1 Paul M. Shiundu Introductory Chemistry 1 Foreword The African Virtual University (AVU) is proud to participate in increasing access to education in African countries through the production of quality learning materials. We are also proud to contribute to global knowledge as our Open Educational Resources (OERs) are mostly accessed from outside the African continent. This module was prepared in collaboration with twenty one (21) African partner institutions which participated in the AVU Multinational Project I and II. From 2005 to 2011, an ICT-integrated Teacher Education Program, funded by the African Development Bank, was developed and offered by 12 universities drawn from 10 countries which worked collaboratively to design, develop, and deliver their own Open Distance and e-Learning (ODeL) programs for teachers in Biology, Chemistry, Physics, Math, ICTs for teachers, and Teacher Education Professional Development. Four Bachelors of Education in mathematics and sciences were developed and peer-reviewed by African Subject Matter Experts (SMEs) from the participating institutions. A total of 73 modules were developed and translated to ensure availability in English, French and Portuguese making it a total of 219 modules. These modules have also been made available as Open Educational Resources (OER) on oer.avu.org, and have since then been accessed over 2 million times. In 2012 a second phase of this project was launched to build on the existing teacher education modules, learning from the lessons of the existing teacher education program, reviewing the existing modules and creating new ones. This exercise was completed in 2017. On behalf of the African Virtual University and our patron, our partner institutions, the African Development Bank, I invite you to use this module in your institution, for your own education, to share it as widely as possible, and to participate actively in the AVU communities of practice of your interest. -
Inorganic Chemistry-Ii
BSCCH- 201 B. Sc. II YEAR INORGANIC CHEMISTRY-II SCHOOL OF SCIENCES DEPARTMENT OF CHEMISTRY UTTARAKHAND OPEN UNIVERSITY BSCCH-201 INORGANIC CHEMISTRY-II SCHOOL OF SCIENCES DEPARTMENT OF CHEMISTRY UTTARAKHAND OPEN UNIVERSITY Phone No. 05946-261122, 261123 Toll free No. 18001804025 Fax No. 05946-264232, E. mail [email protected] htpp://uou.ac.in Board of Studies Prof. Govind Singh Prof. B. S. Saraswat Director, School of Sciences Professor Chemistry Uttarakhand Open University Department of Chemistry School of Sciences, IGNOU, New Delhi Prof S. P. S. Mehta Prof. D. S. Rawat Professor Chemistry Professor Chemistry Department of Chemistry Department of Chemistry DSB Campus, Kumaun University Delhi University, Delhi Nainital Dr. Charu C. Pant Programme Coordinator Department of Chemistry School of Sciences, Uttarakhand Open University Haldwani, Nainital Programme Coordinators Dr. Shalini Singh (Assistant Professor) Department of Chemistry School of Sciences, Uttarakhand Open University Haldwani, Nainital Unit Written By Unit No. 1. Dr. K. S. Dhami (Ret. Proff.) 01, 02, 03, 04 & 05 Department of Chemistry D.S.B. Campus, Kumaun University Nainital 2. Dr. Geeta Tiwari 06, 07, 08 & 09 Department of Chemistry D.S.B. Campus, Kumaun University Nainital Course Editor Prof. B.S. Saraswat Professor of Chemistry (Retd.) School of Sciences, Indira Gandhi National Open University (IGNOU), Maidan Garhi, New Delhi - 110068 Title : Inorganic Chemistry II ISBN No. : 978-93-90845-04-0 Copyright : Uttarakhand Open University Edition : 2021 Published by : Uttarakhand -
Page 1 Stockland Green School
Stockland Green School Q1. The periodic table on the Data Sheet may help you to answer these questions. (a) Part of the periodic table is shown below. The letters are not the symbols of the elements. Choose your answers only from the letters shown in the periodic table above. Which letter, A, B, C, D, E or F, represents (i) aluminium (1) (ii) a Group 5 element (1) (iii) an alkali metal (1) (iv) the element with atomic (proton) number of 47 (1) Page 1 Stockland Green School (v) an element with seven electrons in its outer shell? (1) (b) The table shows the boiling points of the Group 7 elements. The elements are arranged in alphabetical order. Group 7 element Name Symbol Boiling point in °C Astatine At 337 Bromine 58 Chlorine Cl -34 Fluorine F -188 Iodine I 184 (i) The symbol for bromine is missing from the table. What is the symbol for bromine? Symbol = .............................. (1) (ii) Arrange these elements in order of decreasing boiling point. The first one and the last one have been done for you. At ............ ............ ............ F Highest boiling point Lowest boiling point (1) (c) The table shows some statements about Group 7 elements. Page 2 Stockland Green School Tick ( ) the two correct statements. Tick ( ) They are halogens. They are metals. They become less reactive down Group 7. They are compounds. (2) (Total 9 marks) Q2. The elements in Group 1 are known as the alkali metals. Which three of the following are properties of alkali metals? Place a tick ( ) in the box against each correct property.