Geomorphological Hazards in Austria
Total Page:16
File Type:pdf, Size:1020Kb
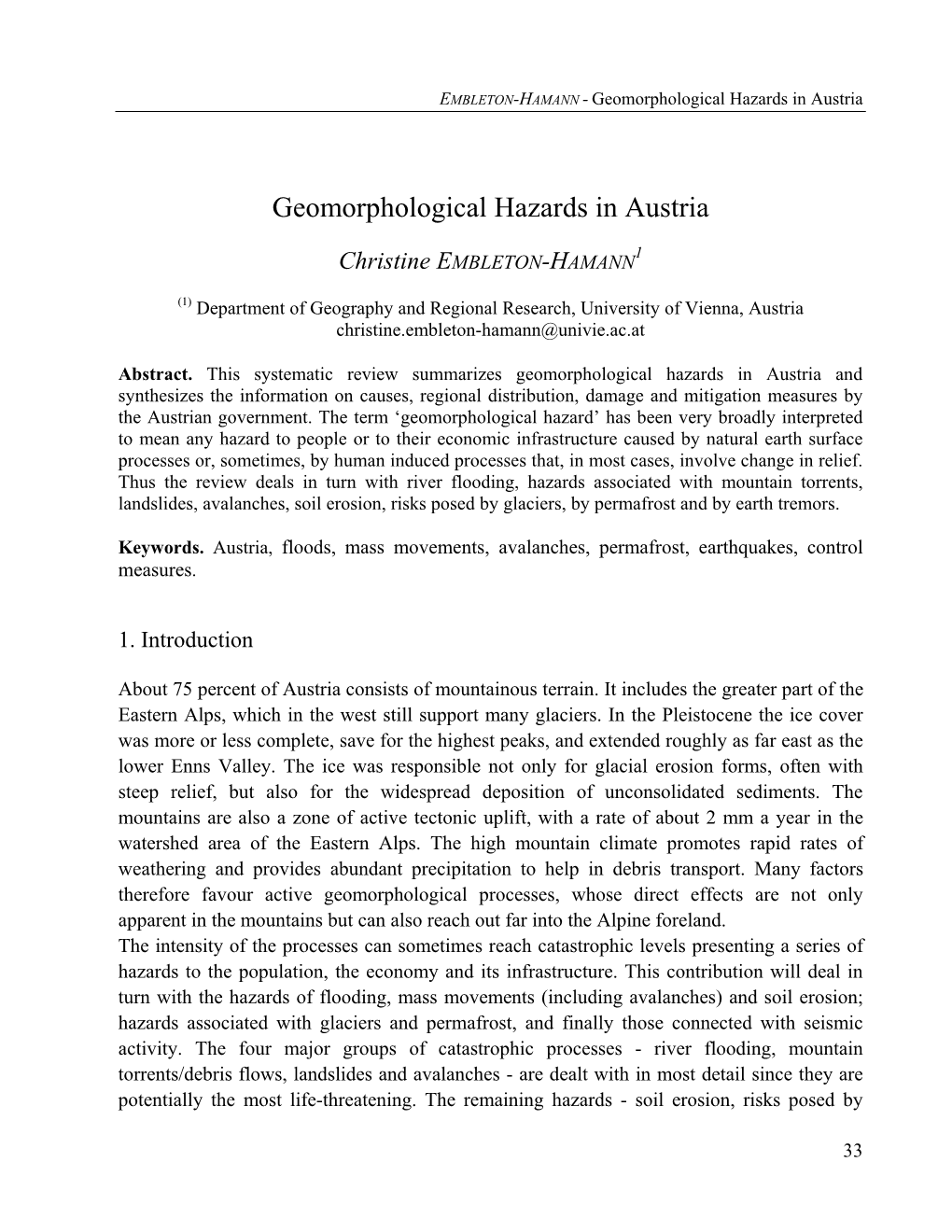
Load more
Recommended publications
-
Chasmophytic Vegetation of Silicate Rocks on the Southern Outcrops of the Alps in Slovenia
ZOBODAT - www.zobodat.at Zoologisch-Botanische Datenbank/Zoological-Botanical Database Digitale Literatur/Digital Literature Zeitschrift/Journal: Wulfenia Jahr/Year: 2011 Band/Volume: 18 Autor(en)/Author(s): Juvan Nina, Carni [ÄŒarni] Andraz [Andraž], Jogan Nejc Artikel/Article: Chasmophytic vegetation of silicate rocks on the southern outcrops of the Alps in Slovenia. 133-156 © Landesmuseum für Kärnten; download www.landesmuseum.ktn.gv.at/wulfenia; www.biologiezentrum.at Wulfenia 18 (2011): 133 –156 Mitteilungen des Kärntner Botanikzentrums Klagenfurt Chasmophytic vegetation of silicate rocks on the southern outcrops of the Alps in Slovenia Nina Juvan, Andraž Čarni & Nejc Jogan Summary: Applying the standard central-European method we studied the chasmophytic vegetation of the silicate rocks on the southern outcrops of the Alps in the territory of Slovenia, in the Kamnik- Savinja Alps, the eastern Karavanke mountains, on Mt. Kozjak and the Pohorje mountains. Three communities of the order Androsacetalia vandellii (Asplenietea trichomanis) were recognized: Campanulo cochleariifoliae-Primuletum villosae ass. nova (Androsacion vandellii ), Woodsio ilvensis-Asplenietum septentrionalis (Asplenion septentrionalis) and Hypno-Polypodietum (Hypno-Polypodion vulgaris). The communities are distinguished by altitude, light intensity, temperature and the number of endemic species, south-European orophytes or cosmopolite species. Altitude is a signifi cant factor aff ecting the fl oristic composition of the studied vegetation. Keywords: chasmophytic -
The American Primrose Society
SOCIETY FOUNDbDlB41 Primroses American Primrose Society Summer 211115 Primroses The Quarterly of the American Primrose Society Volume 65 No 3 SUMMER 2005 The purpose of this Society is to bring the people interested in Primula together in an organization to increase the general knowledge of and interest in the collecting, growing, breeding, showing and using in the landscape and garden of the genus Primula in all its forms and to serve as a clearing house for collecting and disseminating information about Primula. Summer snow in the Alps. The precious Snowbell flowers of a rare white form tfSoldanella minima as photographed by famed alpinist, Franz Hadacek. President's Message, by Ed Buyarski 5 This summer issue of PRIMROSES focuses on Plant Exploration, in Paul Held's Garden - by Amy Olmsted 7 all of it's expressions - from the historically important plant explorers Finding Primroses: Great Plant Explorers by Judith M. Taylor MD () to exploring art in a museum. Ehrct's Auricula by Maedythe Martin 23 In the footsteps of Farrer; Hiking in the Dolomites by Matt Matins 2S PRIMROSES • The Quarterly of the American Primrose Society Editor Editorial Committee Matt Mattus Robert Tonkin 26 Spofford Road Judy Sellers Worcester. MA 01607 Kd Biivarski mmultusfcchartcr.net About the Covers EDITORIAL Manuscripts for publication in the ADVERTISING Advertising rates per issue: full quarterly are invited from members and other page, $100; half page. $50: quarter page, $25; Front Cover: A colony of Primulaceae member Soldanetia alpina, photographed gardeners, although there is no payment. Please eighth page and minimum, SI2.50. Artwork for in Switzerland and kindly submitted by Thomas Huber, Neustadt, Germany. -
Exploring Patterns of Variation Within the Central-European Tephroseris Longifolia Agg.: Karyological and Morphological Study
Preslia 87: 163–194, 2015 163 Exploring patterns of variation within the central-European Tephroseris longifolia agg.: karyological and morphological study Karyologická a morfologická variabilita v rámci Tephroseris longifolia agg. Katarína O l š a v s k á1, Barbora Šingliarová1, Judita K o c h j a r o v á1,3, Zuzana Labdíková2,IvetaŠkodová1, Katarína H e g e d ü š o v á1 &MonikaJanišová1 1Institute of Botany, Slovak Academy of Sciences, Dúbravská cesta 9, SK-84523 Bratislava, Slovakia, e-mail: [email protected]; 2Faculty of Natural Sciences, University of Matej Bel, Tajovského 40, SK-97401 Banská Bystrica, Slovakia; 3Comenius University, Bratislava, Botanical Garden – detached unit, SK-03815 Blatnica, Slovakia Olšavská K., Šingliarová B., Kochjarová J., LabdíkováZ.,ŠkodováI.,HegedüšováK.&JanišováM. (2015): Exploring patterns of variation within the central-European Tephroseris longifolia agg.: karyological and morphological study. – Preslia 87: 163–194. Tephroseris longifolia agg. is an intricate complex of perennial outcrossing herbaceous plants. Recently, five subspecies with rather separate distributions and different geographic patterns were assigned to the aggregate: T. longifolia subsp. longifolia, subsp. pseudocrispa and subsp. gaudinii predominate in the Eastern Alps; the distribution of subsp. brachychaeta is confined to the northern and central Apennines and subsp. moravica is endemic in the Western Carpathians. Carpathian taxon T. l. subsp. moravica is known only from nine localities in Slovakia and the Czech Republic and is treated as an endangered taxon of European importance (according to Natura 2000 network). As the taxonomy of this aggregate is not comprehensively elaborated the aim of this study was to detect variability within the Tephroseris longifolia agg. -
Geologica Ultraiectina
GEOLOGICA ULTRAIECTINA Mededelingen van het Geologisch Instituut der Rijksuniversiteit te Utrecht GRAVITY TECTONICS, GRAVITY FIELD, AND PALAEOMAGNETISM IN NE-ITALY. (With special reference to the Carnian Alps, north of the Val Fella-Val Canale area between Paularoand Tarvisio· Province of Udine-). t I. 34 No. 1 Boer, J.C. den, 1957: Etude g~ologique et paleomagn~tique des Montagnes du Coiron, Ardeche, France No. 2 Landewijk, J.E.J.M. van, 1957: Nomograms for geological pro- blems (with portfolio of plates) No. 3 Palm, Q.A., 1958: Les roches cristalline des C~vennes m~dianes a hauteur de Largentiere, Ardeche, France No. 4 Dietzel, G.F.L., 1960: Geology and permian palaeomagnetism of the Merano Region, province of Bolzano, N. Italy No. 5 Hilten, D. van, 1960: Geology and permian palaeomagnetism of the Val-di-Non Area, W. Dolomites, N. Italy No. 6 Kloosterman, 1960: Le VoIcanisme de la Region D'Agde (Herault France) No. 7 Loon, W. E. van, 1960: Petrographische und geochemische Unter- suchungen im Gebiet zwischen RemUs (Unterengadin) und Nauders (Tirol) Agterberg, F. P., 1961: Tectonics of the crystalline Bas'_ment of the Dolomites in North Italy Kruseman, G.P., 1962: Etude pal~omagn~tique et s~dimentolo- gique du bassin permien de Lodeve, H~rault, France Boer, J. de, 1963: Geology of the Vicentinian Alps (NE-Italy) (with special reference to their palaeomagnetic history) Linden,W.J.M. van der, 1963: Sedimentary structures and facies interpretation of some molasse deposits Sense -Schwarzwasser area- Canton Bern, Switzerland Engelen, G. B. 1963: Gravity tectonics of the N. Western Dolo- mites (NE Italy). -
Lungauer Winterbonus Card Damit Ihr Urlaub Garantiert Zu Einem Unvergesslichen Erlebnis Wird
Lungauer Winterbonus Card Damit Ihr Urlaub garantiert zu einem unvergesslichen Erlebnis wird. Attraktive Freizeitangebote, Museen, Wellness, Kulinarik, Sportmöglichkeiten und vieles mehr. Für alle Gäste kostenlos in der Urlaubsregion Mauterndorf und Mariapfarr, Tamsweg, St. Andrä, Ramingstein und Lessach! TOURISMUSVERBAND MAUTERNDORF 5570 Mauterndorf, Österreich Tel.: +43 (0)6472 / 7949 E-Mail: [email protected] Lungauer www.mauterndorf.at Winterbonus Card TOURISMUSVERBAND MARIAPFARR 5571 Mariapfarr, Österreich Damit Ihr Urlaub garantiert Tel.: +43 (0)6473 / 8766 zu einem unvergesslichen Erlebnis wird. E-Mail: [email protected] www.mariapfarr.at www.mauterndorf-mariapfarr.at www.tourismuslungau.at TOURISMUSVERBAND TOURISMUS LUNGAU 5580 Tamsweg, Österreich Tel.: +43 (0)6474 / 2145 E-Mail: [email protected] www.tourismuslungau.at Gratisleistungen Benützung aller Loipen in Weißpriach Benützung der Preber Rodelbahn Benützung aller Loipen in Mauterndorf Benützung der Rodelbahn und Schlitten bei der Wildbachhütte (Lessach) Benützung der Taurachloipe in St. Andrä Benützung des Eislaufplatzes (Kein Schuhverleih) im Sportzentrum Tamsweg Benützung der Loipe Lessach (nur bei entsprechender Witterung) Benützung der Höhenloipe Prebersee (Tamsweg) Benützung des Eislaufplatzes in Ramingstein (Nur Mo. bis Fr. von 11.00 bis Benützung der Loipe Lignitz und der täglich beleuchteten Nacht-Langlaufloipe 16.00 Uhr - nur bei entsprechender Witterung) im Langlaufzentrum Lignitz 1 x Kennenlernen des Eisstocksportes auf der Eisstockbahn mit Leihstock -
Technical Note About the Monitoring of Hydromorphological Restoration/Management of the Drau River (Carinthia, Austria)
Technical note about the monitoring of hydromorphological restoration/management of the Drau River (Carinthia, Austria) BOKU 1 1. General presentation of the study site The study site at the Austrian Drau River is located in the Upper Drau valley in the South of Austria in the region of Carinthia, mainly focussing on two restored reaches near the villages of Kleblach (Figure 1a) and Obergottesfeld. This area is part of the Austrian Alps; elevations reach from around 550 m up to more than 3000 m. The catchment basin drains around 2445 km² and covers parts of the southern limestone Alps as well as east alpine crystalline. The dominant sediment is gravel with a d50 diameter of 25 mm. Sediment input mainly results from upstream active torrents in the catchment. The channel slope at the study site is approximately 0.002. The mean discharge is about 74 m³/s; a one-year flood reaches 320m³/s. Floods mostly occur in spring when snowmelt – and also glacier melt - is released into the basin, or in summer after thunderstorms. The discharge regime can be described as strongly pronounced nivo-glacial with a maximum discharge in June (Mader, 1996). The mean annual rainfall in Sachsenburg (close to the study site) is around 982 mm/a (BMFLUW, 2014). Historically, the Drau River at the studied reach was a wandering river (Figure 1b), which results from a transition from a braided to a meandering morphology, before it was regulated and finally restored. At the study site the valley is unconfined with presence of an alluvial forest whereas most parts of the Drau River are straightened and narrowed with a narrow alluvial forest zone left. -
Official Journal C 195 of the European Union
Official Journal C 195 of the European Union Volume 61 English edition Information and Notices 7 June 2018 Contents I Resolutions, recommendations and opinions RECOMMENDATIONS Council 2018/C 195/01 Council Recommendation of 22 May 2018 on promoting common values, inclusive education, and the European dimension of teaching ........................................................................................ 1 II Information INFORMATION FROM EUROPEAN UNION INSTITUTIONS, BODIES, OFFICES AND AGENCIES European Commission 2018/C 195/02 Non-opposition to a notified concentration (Case M.8887 — Platinum Equity/LifeScan) (1) ................ 6 2018/C 195/03 Non-opposition to a notified concentration (Case M.8771 — Total/Engie (Part of Liquefied Natural Gas Business)) (1) .................................................................................................................. 6 EN (1) Text with EEA relevance. IV Notices NOTICES FROM EUROPEAN UNION INSTITUTIONS, BODIES, OFFICES AND AGENCIES Council 2018/C 195/04 Council conclusions on moving towards a vision of a European Education Area .............................. 7 2018/C 195/05 Council conclusions on the role of young people in building a secure, cohesive and harmonious society in Europe ............................................................................................................................... 13 European Commission 2018/C 195/06 Euro exchange rates ............................................................................................................. -
Ergebnisse Knaben Kategorie Ia Rang Startnr
Ergebnisse Knaben Kategorie Ia Rang Startnr. Name Schule 1 1 JESSNER David VS St.Andrä 2 22 SCHLICK David VS Oberweissburg 3 32 RAUTER Fabio VS Zederhaus 4 18 MAIER Johannes VS Mauterndorf 5 41 BAUER Matthias VS Mariapfarr 6 14 KORNTNER Bastian VS St.Michael 7 21 GRUBER Jakob VS Oberweissburg 8 4 ANTRETTER Jakob VS Tamsweg 9 31 SCHLICK Elias VS Zederhaus 10 5 HASANOVIC Jan Benjamin VS Tamsweg 12 15 PETZLBERGER Ulrich VS St.Michael 13 37 PRODINGER Sebastian VS Mariapfarr 14 38 MACHEINER Christoph VS Mariapfarr 15 45 HOLZER Sebastian VS Mariapfarr 16 12 DOPPLER Tobias VS Lessach 17 10 SCHMID Noah VS Tamsweg 18 40 CERRETO Emanuele VS Mariapfarr 19 47 SANTNER Emilio VS Mariapfarr 20 30 GFRERER Finn VS Zederhaus 21 36 BRUGGER Lukas VS Mariapfarr 22 13 JESSNER Michael VS Lessach 23 17 MITTERWALLNER Alexander VS Tweng 24 6 KÖNIG Konstantin VS Tamsweg 25 46 KARNER Lukas VS Mariapfarr 26 19 KESSLER Paul VS Mauterndorf 27 23 BAYER Erik VS Weisspriach 28 20 SPREITZER Max VS Mauterndorf 29 24 BAYER Philipp VS Weisspriach 30 26 PERTL Peter VS Weisspriach 31 48 SCHIEFER Vincent VS Mariapfarr 32 42 BINGGL Thomas VS Mariapfarr 33 33 BLIEM Lukas VS Zederhaus 34 50 WIELAND Rupert VS Mariapfarr 35 35 SCHREILECHNER Martin VS Mariapfarr 36 29 SCHLICK Andreas VS Zederhaus 37 44 GRAGGABER Felix VS Mariapfarr 38 49 SEIFTER Samuel VS Mariapfarr 39 11 WIELAND Nicolas VS Tamsweg 40 2 FEUCHTER Tobias VS St.Andrä 41 25 LASSACHER Noah VS Weisspriach 42 43 BOGENSPERGER Fabian VS Mariapfarr 43 27 PRODINGER Sebastian VS Weisspriach 44 39 KOCHER David VS Ramingstein 45 28 SCHIEFER Maximilian VS Weisspriach 46 8 MOSER Maximilian VS Tamsweg 47 9 GRGIC Deniz VS Tamsweg N.i.Z. -
Administrative Units of the Alpine Convention Alpine the of Units Administrative Alpine Signals 1 Signals Alpine 21
Administrative Units of the Alpine Convention Administrative Units Alpine signals 1 21 Scope of application of the Alpine Convention Administrative Units LIST OF ADMINistrative UNITS OF THE ALPINE CONVENTION IN 1) According to the Federal Official Journal (of the Republic of Austria) THE REPUBLIC OF AUSTRIA III vol. 18/1999 from 01.28.1999. Federal state of Strobl Weißpriach VORARLBERG Thalgau Zederhaus all municipalities Wals-Siezenheim District of Zell am See F e d e r a l s t a t e o f T Y R O L District of Sankt Johann im Pongau Bramberg am Wildkogel all municipalities Altenmarkt im Pongau Bruck an der Großglockner- straße Bad Hofgastein Federal state of Dienten am Hochkönig CARINTHIA Badgastein Bischofshofen Fusch an der Großglockner- all municipalities straße Dorfgastein Hollersbach im Pinzgau Eben im Pongau Federal state of Kaprun SALZBURG Filzmoos Flachau Krimml Lend Salzburg (town area) Forstau Goldegg Leogang District of Hallein Großarl Lofer Hüttau Maishofen Abtenau Maria Alm am Steinernen Adnet Hüttschlag Kleinarl Meer Annaberg im Lammertal Mittersill Golling an der Salzach Mühlbach am Hochkönig Pfarrwerfen Neukirchen am Großvene- Hallein diger Krispl Radstadt Sankt Johann im Pongau Niedernsill Kuchl Piesendorf Oberalm Sankt Martin am Tennen- gebirge Rauris Puch bei Hallein Saalbach-Hinterglemm Rußbach am Paß Gschütt Sankt Veit im Pongau Schwarzach im Pongau Saalfelden am Steinernen Sankt Koloman Meer Scheffau am Tennengebirge Untertauern Sankt Martin bei Lofer Vigaun Wagrain Stuhlfelden District Werfen Taxenbach Salzburg/Surrounding -
Alpine Summer with the FRIENDLY ASSISTANCE of ASSISTANCE the FRIENDLY WITH
Salzburg‘s Lungau region Information General at a glance Alpine Summer Fabulous Hikes to Alpine Tarns Tarns Hikes to Alpine Fabulous Tours from Lake to Mountain from Tours Flavor of the High Country Flavor Hut and mountain lake hiking in Salzburg‘s Lungau region Ferienregion Salzburger Lungau Parks & Nature Trails Theme Rotkreuzgasse 100 A-5582 St. Michael T +43 (0)6477 8988 F +43 (0)6477 8988-20 [email protected] www.lungau.at WITH THE FRIENDLY ASSISTANCE OF 101 Table of Contents Alpine tarns and huts in Salzburg‘s Lungau region General Information Page 3 Fabulous Hikes to Alpine Tarns Page 4 Tours from Lake to Mountain Lake Page 28 Flavor of the High Country Page 45 General Information General Theme Trails & Nature Parks Page 95 In conclusion, the Salzburg’s Lungau Holiday Region wishes to thank: • Dr. Gertraud Steiner for authoring the editorial texts, • Mr. Peter Bayr for his descriptions of the alpine hut and lake routes, as well as for compiling cartographical materials, • Mr. Hannes Modl for his descriptions of the alpine hut and lake routes, as well as for his wealth of ideas during the creative and realization process, and also • all of the owners, managers and staff of our region’s alpine huts. Without their support and dedication, it would not have been possible to produce this brochure. Additional Reading on this Topic: Fabulous Hikes to Alpine Tarns Tarns Hikes to Alpine Fabulous • Dr. Gertraud Steiner: Winkelwelt. Sagen aus dem Lungau. Wolfgang Pfeifenberger Verlag 1999 • Dr. Gertraud Steiner: Sagen und Mythen entdecken auf Salzburger Almen. -
European Rural Futures
European Rural Futures This project is implemented through the CENTRAL EUROPE Programme co-financed by the ERDF 1 Editor LEAD PARTNER EURUFU Thuringian Ministry for Building, Regional Development and Infrastructure Service Agentur Demografischer Wandel und EU Projekte Dr. Klaus Bongartz Werner-Selenbinder-Straße 8, D-99096 Erfurt Tel.: +49 (0)361 / 3791515; Fax: +49 (0)361 / 3791399 Mail: [email protected] THE PROJECT CONSORTIUM Germany Unioversity of Applied Sciences Erfurt, Institut für Verkehr und Raum Czech Republic Region Usti nad Labem Austria Salzburg Institut for Spatial Planning Regionalmanagement Upper Styria West Hungary Central Transdanubian Regional Development Agency Office for National Economic Planning Italy Development Agency Langhe Monferrato Roero Slovenia Regional Development Agancy SORA Biotechnical Center Naklo Polen Municipality Brzeg Dolny PROJECT Duration: 05/2011 – 04/2014 Budget: 2. 163. 713 EURO EFRE: 1. 720. 938 EURO 2 www.eurufu.eu Preface Demographic change is not a political fad, but a tangible socio-political challenge for Thuringia and Europe. Demographic developments induce changes in society, which influence the face of our continent. Population decline, growing proportion of older EU citizens and strong regional differences in the development provide us with major challenges. These must be tackled and opportunities must be exploited courageously. Central Issues for the Future are the maintenance and development of technical and social infrastructure, especially in rural regions. Answers to this question affect not only individual parts of the country or states but the European Union as a whole. The EU itself has adopted the Strategy “EU 2020” and thereby set the objectives and tools to make all European regions fit for the future. -
Journal 25.Indb
POLISH JOURNAL OF ECOLOGY 59 1 153–163 2011 (Pol. J. Ecol.) Regular research paper Paweł KOPERSKI1, Elżbieta DUMNICKA2*, Joanna GALAS2 1 Department of Hydrobiology, Warsaw University, Banacha 2, 02-095 Warszawa, Poland, e-mail: [email protected] 2 Institute of Nature Conservation, Polish Academy of Sciences, Mickiewicza 33, 31-120 Kraków, Poland, *e-mail: [email protected] (corresponding author) ABIOTIC PARAMETERS DETERMINING FAUNA COMPOSITION IN KARSTIC SPRINGS ABSTRACT: The biotic diversity of springs mentioned above had other abiotic determinants is specific, which makes them valuable sites im- such as alkalinity, NO3 and temperature. portant for nature protection. Springs located in the Krakow-Częstochowa Upland (southern Po- KEY WORDS: macroinvertebrates, multi- land) are characterized by low variability of en- variate analysis, springs, crenobiology, Poland vironmental conditions, but their benthic fauna composition is considerably different. Benthic 1. INTRODUCTION invertebrates, water chemistry as well as sediment characteristics of 25 springs were studied four Springs are sensitive to various kinds of times in 2003. The relationships between fauna anthropogenic changes (encasing, tourist composition and abiotic parameters were ascer- impact, water exploitation etc.) which dep- tained using multivariate statistical analyses. In total, fifty families or subfamilies and four higher redate unique benthic fauna communities by taxa of invertebrates were identified in the springs destroying their habitats. Springs in Poland studied. Only Gammarus fossarum (Amphipoda) are usually protected as inanimate nature occurred in all of the springs, whereas crenophilic monuments (Baścik 2004) but the diversity taxonomic groups such as Turbellaria, Bythinel- of their benthic fauna and the occurrence of linae, Nemouridae, Limoniidae, Limnephilidae rare or endangered species have not been tak- and Enchytraeidae as well as ubiquitous taxa such en into account.