Paul Macdonald Magyar
Total Page:16
File Type:pdf, Size:1020Kb
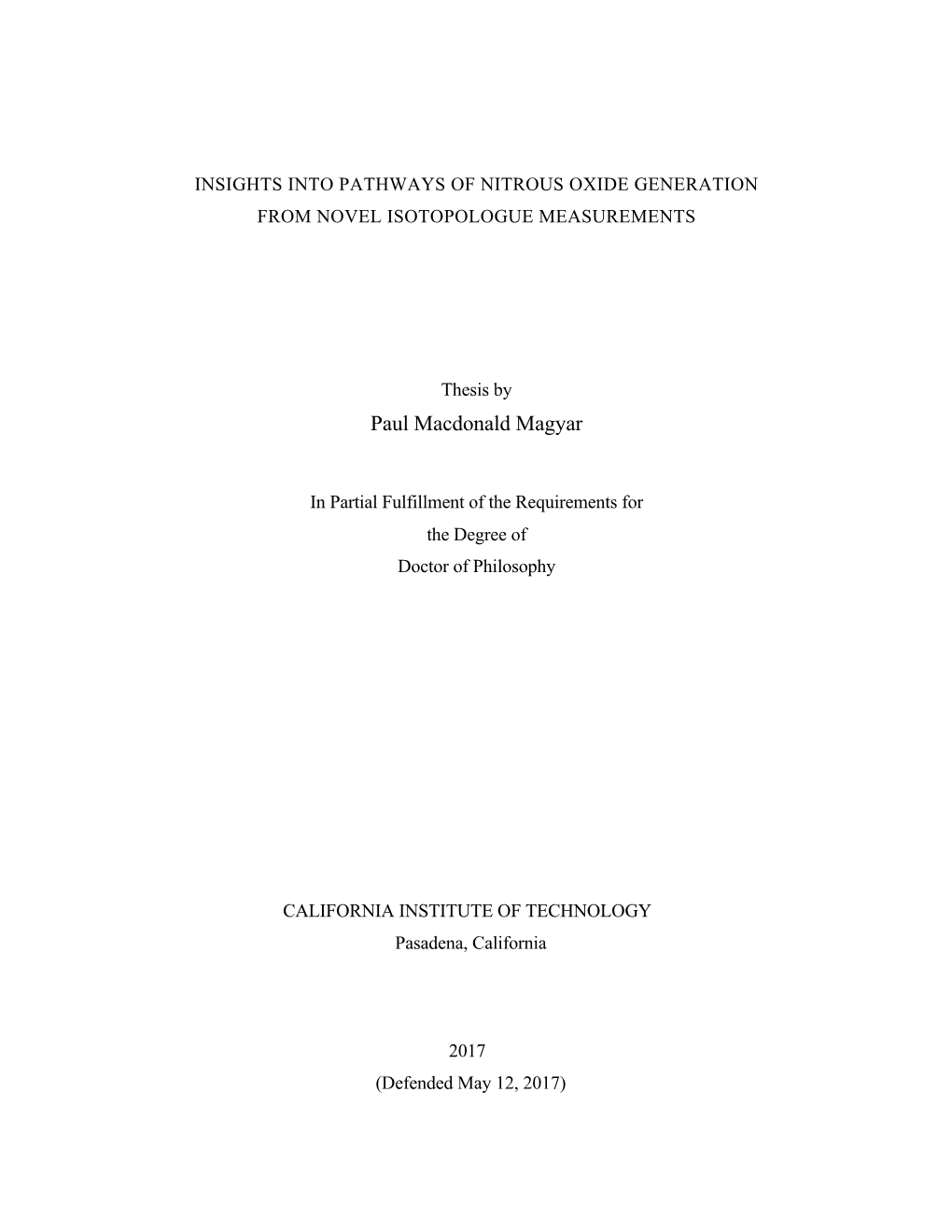
Load more
Recommended publications
-
Diving Deep for Knowledge Stars in Our Solar System
The Daring Journey “ The exhilaration of seeing first- ’m an explorer at heart. As a child I was al- ways drawn to the ocean and the mysterious hand previously unexplored areas of Ianimal life below its surface. As an adult I’m the deep ocean and the promise for still captivated by the ocean’s vast and powerful life-sustaining role on Earth, but I’ve turned my new discoveries remains as strong attention away from the charismatic tropical fish, a motivator for me today as on my coral reefs and marine mammals that captured my imagination in my youth to a vibrant ocean first dive well over a decade ago.” world of microbes that often goes unnoticed. This world is invisible to our unaided eye — teeming with single-celled bacteria, archaea, protists and viruses, the smallest life forms and among the most difficult to study. They number among the million trillion trillions in ocean waters and deep sediments, far surpassing the total number of Diving Deep for Knowledge stars in our solar system. Researchers in the relatively young field known as geobiology are just beginning to understand the intricacies of this complex microbial world closely with organic and isotope geochemists, pe- associated with each of our respective methodolog- and how it has changed over the course of Earth’s troleum geologists and earth scientists. From this ical approaches was a challenge. I still remember history. However, what is clear is that the col- experience, I gained a deep appreciation for the my dismay after discussing the details of the SIMS lective metabolic activity of microorganisms in benefits and challenges of cross-disciplinary col- instrument with Chris over the phone, having real- terrestrial and marine environments continues to laborations. -
CURRICULUM VITAE Edward Francis Delong, Professor Department Of
CURRICULUM VITAE Edward Francis DeLong, Professor Department of Oceanography, School of Ocean and Earth Science and Technology Center for Microbial Oceanography: Research and Education Co-Director, Simons Collaboration on Ocean Processes and Ecology University of Hawaii, Manoa, Honolulu, 96822 Ph: 808-956-0563 Fax: 808-956-5059 email: [email protected]; [email protected] http://en.wikipedia.org/wiki/Edward_DeLong http://scholar.google.com/citations?user=wEkWbTQAAAAJ&hl=en http://scope.soest.hawaii.edu http://www.soest.hawaii.edu/oceanography/faculty/DeLong.html Education: A. S., Biology, Santa Rosa Junior College, 1980 B. S., Bacteriology, University of California Davis, 1982 Ph. D., Marine Biology, Scripps Institute of Oceanography, 1986 Thesis title: Adaptations of deep-sea bacteria to the abyssal environment Professional Experience: 2014- Professor of Oceanography, SOEST, University of Hawaii, Manoa 2014- co-Director, Simons Collaboration on Ocean Processes and Ecology (SCOPE) 2006-2016 co-Director, Center for Microbial Oceanography: Research and Education 2004-2015 Professor, Massachusetts Institute of Technology 1998-2004 Senior Scientist V, Monterey Bay Aquarium Research Inst. 1997-2002 Science Dept. Chair, Monterey Bay Aquarium Research Inst. 1997-1999 Associate Scientist III, Monterey Bay Aquarium Research Inst. 1996-1998 Associate Professor, Ecology, Evolution Marine Biol. Dept., UCSB 1992-1996 Assistant Professor, Biology Dept., UC Santa Barbara 1989-1991 Assistant Scientist, Woods Hole Oceanographic Inst. 1986-1989 Post-doctoral Research Assoc., Indiana U., Bloomington, Indiana 1984-1986 Teaching Assistant, Biology Dept., UC San Diego 1982-1986 Research Assistant, Scripps Inst. of Oceanography, UC San Diego 1981-1982 Research Assistant, University of California, Davis, Ca. Professional interests: Physiology, biochemistry, ecology, and evolution of microbes. -
Seeing Protein Synthesis in the Field 8 September 2014, by Ramanuj Basu
Seeing protein synthesis in the field 8 September 2014, by Ramanuj Basu BONCAT uses "non-canonical" amino acids—synthetic molecules that do not normally occur in proteins found in nature and that carry particular chemical tags that can attach (or "click") onto a fluorescent dye. When these artificial amino acids are incubated with environmental samples, like lily-pond water, they are taken up by microorganisms and incorporated into newly formed proteins. Adding the fluorescent dye to the mix allows these proteins to be visualized within the cell. For example, in the image, the entire microbial community in the pond water is stained blue with a DNA dye; freshwater gammaproteobacteria are labeled with a fluorescently tagged short-chain ribosomal RNA probe, in red; and newly created In this image, the entire microbial community in proteins are dyed green by BONCAT. The cells Caltech's lily-pond water is stained blue with a DNA dye; colored green and orange in the composite image, freshwater gammaproteobacteria are labeled with a fluorescently tagged short-chain ribosomal RNA probe, then, show those bacteria—gammaproteobacteria in red; and newly created proteins are dyed green by and other rod-shaped cells—that are actively making BONCAT. The green and orange cells in the composite proteins. image (lower right) show gammaproteobacteria and other rod–shaped cells that are actively making proteins. "You could apply BONCAT to almost any type of Credit: Roland Hatzenpichler and Victoria sample," Orphan says. "When you have an Orphan/Caltech environmental sample, you don't know which microorganisms are active. So, assume you're interested in looking at organisms that respond to methane. -
BIO-Annual-Report-2012.Pdf
The Annual Report of the Division of Biology at the California Institute of Technology (Caltech) presents major research accomplishments of Caltech's faculty, students and staff. Through their Principle Investigators, each Caltech group, laboratory, or center submits a report on research projects that were active during the prior Caltech academic year. These reports summarize research progress and results, and list personnel, sponsors, and their publications. The 2012 report covers the time period: July 1, 2011 - June 30, 2012. For more information on the Biology Annual Report please contact Julie Boucher Caltech Division of Biology 1200 East California Blvd MC 156-29 Pasadena, CA 91125 (626) 395-4952 [email protected] T ABLE OF C ONTENTS 2012 Click on title to view page, when done click logo at bottom to go back to table of contents. 2011-2012 News, Events and People 6 Press Releases 10 New Faculty Members 11 Annual Biology Retreat 14 Ferguson Prize 15 Professorial Awards and Honors 17 Division of Biology Seminars 21 Named Lectures 22 Biology Graduate Students 23 Biology Graduates 26 Financial Support and Donors 28 Faculty and Research Staff 34 Administrative Staff Molecular, Cellular, and Integrative Neuroscience 38 Adolphs, Ralph - Bren Prof. of Psychology and Neuroscience, Prof. of Biology 42 Allman, John - Frank P. Hixon Prof. of Neurobiology 44 Andersen, Richard - James G. Boswell Prof. of Neuroscience 48 Anderson, David - Seymour Benzer Prof. of Biology 52 Koch, Christof - Lois and Victor Troendle Prof. of Cognitive and Behavioral Biology and Prof. of Computation and Neural Systems 56 Konishi, Masakazu - Bing Prof. of Behavioral Biology 58 Lester, Henry - Bren Prof. -
Symbiotic Diversity and Mineral-Associated Microbial Ecology in Marine Microbiomes
Symbiotic diversity and mineral-associated microbial ecology in marine microbiomes Thesis by Kyle Shuhert Metcalfe In Partial Fulfillment of the Requirements for the degree of Doctor of Philosophy CALIFORNIA INSTITUTE OF TECHNOLOGY Pasadena, California 2020 (Defended August 3, 2020) i © 2020 Kyle Shuhert Metcalfe ORCID: 0000-0002-2963-765X ii ACKNOWLEDGEMENTS This thesis would not have been possible if not for the opportunities afforded by my thesis advisor Victoria Orphan. Thank you, Victoria, for your inspiring drive to push the boundaries of microbial ecology, and for your boundless energy to take on any project no matter how challenging. It has been a privilege to pursue my doctorate in the Orphan Lab and under its aegis dive three thousand feet beneath the western Pacific on Alvin and drill nineteen thousand feet beneath the eastern Pacific on Chikyu. I cannot be thankful enough for your tireless work that made this thesis possible. I would like to thank Woody Fischer for his ability to bring fresh, exciting ideas into any research question and for always pushing me to become a better scientist. The weekly meetings of the Geobiology Reading Group were a highlight of my academic career at Caltech and profoundly expanded my perspective on the breadth of disciplines within Geobiology. I also am thankful for Woody’s advice and guidance in the project that became Chapter 4 of this thesis and for his thoughtful comments on the other chapters. I’d like to thank both Woody and John Grotzinger for leading expeditions to the Turks and Caicos Islands and for assembling such an incredible team to explore the microbiology and sedimentology of Little Ambergris Cay. -
NASA ASTROBIOLOGY STRATEGY 2015 I
NASA ASTROBIOLOGY STRATEGY 2015 i CONTRIBUTIONS Editor-in-Chief Lindsay Hays, Jet Propulsion Laboratory, California Institute of Technology Lead Authors Laurie Achenbach, Southern Illinois University Karen Lloyd, University of Tennessee Jake Bailey, University of Minnesota Tim Lyons, University of California, Riverside Rory Barnes, University of Washington Vikki Meadows, University of Washington John Baross, University of Washington Lucas Mix, Harvard University Connie Bertka, Smithsonian Institution Steve Mojzsis, University of Colorado Boulder Penny Boston, New Mexico Institute of Mining and Uli Muller, University of California, San Diego Technology Matt Pasek, University of South Florida Eric Boyd, Montana State University Matthew Powell, Juniata College Morgan Cable, Jet Propulsion Laboratory, California Institute of Technology Tyler Robinson, Ames Research Center Irene Chen, University of California, Santa Barbara Frank Rosenzweig, University of Montana Fred Ciesla, University of Chicago Britney Schmidt, Georgia Institute of Technology Dave Des Marais, Ames Research Center Burckhard Seelig, University of Minnesota Shawn Domagal-Goldman, Goddard Space Flight Center Greg Springsteen, Furman University Jamie Elsila Cook, Goddard Space Flight Center Steve Vance, Jet Propulsion Laboratory, California Institute of Technology Aaron Goldman, Oberlin College Paula Welander, Stanford University Nick Hud, Georgia Institute of Technology Loren Williams, Georgia Institute of Technology Pauli Laine, University of Jyväskylä Robin Wordsworth, Harvard -
MMI Evaluation Summary Report June 2013
SUMMARY1 OF AAAS 2012 EVALUATION OF THE GORDON AND BETTY MOORE FOUNDATION’S MARINE MICROBIOLOGY INITIATIVE, PHASE 1 (2004–2010) BACKGROUND Oceans account for ~70% of Earth’s surface. Primary productivity, many chemical cycles, and food webs depend on the ocean’s microorganisms, which also convert carbon, nitrogen, sulfur, and trace minerals like iron from inorganic to biologically usable forms. We know that these important marine systems and processes Box 1. Marine microbial ecology defined have been experiencing unprecedented stress due to Marine microbial ecology is the study of increased nutrient run-off, overfishing, and pervasive and the single-celled organisms (bacteria, significant changes in ocean chemistry and temperature archaea, and eukaryotes) and viruses that live in the ocean and their interactions driven by increased emissions of greenhouse gases, yet our with each other and the environment specific knowledge is limited. Despite the fact that research around them. These microorganisms and in this field began more than 75 years ago, as recently as viruses are the ocean’s smallest and most the early 2000s, even the most basic ecological questions— abundant creatures, and they drive many Which organisms are present? What do they do? How do of the chemical reactions that comprise they interact?—remained unanswered due primarily to the Earth’s marine biogeochemical cycles. major obstacles such as: • An inability to grow most microbes in culture in tandem with limited morphological characteristics capable of differentiating species, creating a major taxonomic impediment resulting in treatment of broad groups of microbes as “black boxes” with little knowledge of the role of each species. -
On the Past, Present, and Future Role of Biology in NASA's Exploration Of
Biology and Solar System Exploration Decadal Survey 2023-3032 On the Past, Present, and Future Role of Biology in NASA’s Exploration of our Solar System Dr. Kevin P. Hand, Jet Propulsion Laboratory, California Institute of Technology1, [email protected], Planetary Science, Astrobiology [primary discipline(s)] Dr. Cynthia B. Phillips, Jet Propulsion Laboratory, California Institute of Technology, Planetary Science Professor Christopher F. Chyba, Department of Astrophysics, Princeton University, Planetary Science, Astrobiology Professor Brandy Toner, University of Minnesota - Twin Cities, Biogeochemistry Dr. Kakani Katija, Monterey Bay Aquarium Research Institute, Bioengineering Professor Victoria Orphan, California Institute of Technology, Environmental Microbiology, Geobiology Dr. Julie Huber, Woods Hole Oceanographic Institution, Marine Microbiology Professor Colleen M. Cavanaugh, Dept. of Organismic and Evolutionary Biology, Harvard University, Microbial Ecology/Evolution and Symbiosis Professor Marian Carlson, Director of Life Sciences, Simons Foundation, Professor Emerita of Genetics & Development, Columbia University, Molecular Genetics Professor Brent Christner, University of Florida, Microbiology Professor Alexis Templeton, University of Colorado, Geobiology Dr. Jeffrey Seewald, Woods Hole Oceanographic Institution, Geochemistry Dr. Jason D. Hofgartner, Jet Propulsion Laboratory, California Institute of Technology, Planetary Science Professor Jan P. Amend, Biological Sciences & Earth Sciences Depts., Divisional Dean for Life Sciences, -
California Insɵtute of Technology Division of Biology and Biological
California InsƟtute of Technology Division of Biology and Biological Engineering Annual Report 2017 Introduction Annual Report | Biology and Biological Engineering | 2017 Introduction The annual report for Caltech's Division of Biology and Biological Engineering (BBE) presents major research accomplishments of faculty, students, and staff during the previous academic year. This report covers October 1, 2016 to September 30, 2017. Front Cover Illustration Vector-assisted spectral tracing (VAST) in the cerebellum of an adult mouse A movie highlighting the multi-color vector-assisted spectral tracing (VAST) system in the cerebellum of an adult mouse. Due to the stochastic uptake of AAV-PHP viruses encoding either a blue, green or red fluorescent protein, cells are labeled with a wide range of hues. This approach can be used to differentiate neighboring neurons for morphology and tracing studies. Credit: Ben Deverman et al., Gradinaru Lab Back Cover Illustration Engineered adeno-associated viruses efficiently cross the blood-brain-barrier for enhanced brain transduction in adult mice. Representative images of virally-delivered nuclear GFP fluorescence (green) and Calbindin immunohistochemistry (magenta) in the cerebellum. Credit: Chan et al., Gradinaru Lab 2 News, Events, and People Annual Report | Biology and Biological Engineering | 2017 Press Releases 6 Annual Retreat 15 Ferguson Prize 17 Ferguson Prize 18 Professorial Awards and Honors 19 Seminars 20 Named Lectures 26 3 News, Events, and People Annual Report | Biology and Biological Engineering -
Roland S. Hatzenpichler, Phd
Roland Hatzenpichler Curriculum vitae Roland S. Hatzenpichler, PhD California Institute of Technology Division of Geological and Planetary Sciences 1200 E. California Blvd, MC 100-23, Pasadena, CA-91125 phone: 626-319-2707 [email protected] www.gps.caltech.edu/content/roland-s-hatzenpichler Curriculum vitae Postdoc, current position since July 2012, Marie Curie Fellow, Postdoctoral Scholar in Geobiology Division of Geological and Planetary Sciences (GPS), California Institute of Technology Advisor: Victoria Orphan, Professor of Geobiology July 2011 - June 2012, O.K. Earl Postdoctoral Scholar in Geobiology, GPS Division, Caltech Advisor: Victoria Orphan, Professor of Geobiology Doctor of natural sciences (PhD) May 2006 - June 2011, Department of Microbial Ecology, University of Vienna, Austria Advisor: Michael Wagner, Full Professor for Microbial Ecology Thesis: Identification and characterization of novel ammonia-oxidizing archaea and bacteria Title of qualification: Doctor rerum naturalium (Doctor of natural sciences, PhD) Graduation with perfect grade point average Three year PhD student fellowship (DOC) of the Austrian Academy of Sciences, 2007-2009 Doc.Award for outstanding PhD thesis by the City of Vienna Master of science April 2005 - April 2006, Department of Microbial Ecology, University of Vienna, Austria Advisor: Michael Wagner, Full Professor for Microbial Ecology Thesis: Diversity analyses and in situ detection of nitrifying prokaryotes in hot springs and primeval forest soil Title of qualification: Magister rerum naturalium (Master of natural sciences) Passed with distinction Undergraduate studies October 2001 - April 2005, University of Vienna, Austria Study of microbiology and genetics, with focus on environmental microbiology, molecular microbiology and genetics, as well as analytical and organic chemistry Completion of studies with distinction Additional training June 13 - July 30, 2009, Microbial Diversity course, Marine Biological Laboratory, Woods Hole (MA), USA; Advisors: Associate Prof. -
Diving for Microbes
Sixteen panels of four different clumps of marine microorganisms collected from a methane seep off the coast of northern California. Each clump, called an aggregate, is about 10 microns (millionths of a meter) across. The microbes have been tagged with fluorescent markers. DNA glows blue. Diving for Microbes Off the western coast of Costa Rica, where the crust of the North American continent ends, the underwater terrain is smooth and barren. A thousand meters deep, the seafloor is beyond the reach of the sun and low in oxygen. “It’s just amazing—the bottom of the deep ocean at that site is surprisingly desolate,” says Anne Dekas, a graduate stu- dent in Assistant Professor of Geobiology Victoria Orphan’s lab. “It’s just flat and gray sediment for as long as you can see.” Break- ing the monotony are occasional methane seeps—places where methane trapped in ice (see box) is released and bubbles out through cracks in the seabed—and at these seeps, Dekas says, “it’s completely differ- ent. It’s an oasis of life.” Boasting clams, crabs, tube worms, shrimp, and microbial mats, these dark, deep-sea oases are made possible by microorganisms that eat the bountiful methane, producing nutrients and sources of energy for the rest of the food chain. But these microorganisms do more than just form the foundation of exotic ecosystems—they’re crucial for the entire planet. Methane is a powerful greenhouse gas, about 20 times more adept at trapping In the harsh conditions of the ocean floor, a mysterious medley of heat than carbon dioxide. -
Understanding the Symbiosis in Anaerobic Oxidation of Methane Through Metabolic, Biosynthetic and Transcriptomic Activities
UNDERSTANDING THE SYMBIOSIS IN ANAEROBIC OXIDATION OF METHANE THROUGH METABOLIC, BIOSYNTHETIC AND TRANSCRIPTOMIC ACTIVITIES Thesis by Hang Yu (Hank) In Partial Fulfillment of the Requirements for the degree of Doctor of Philosophy CALIFORNIA INSTITUTE OF TECHNOLOGY Pasadena, California 2017 (Defended on April 26, 2017) ii ã 2017 Hang Yu ORCID: 0000-0002-7600-1582 iii ACKNOWLEDGEMENTS My accomplishments today would not have been possible without the contributions and guidance from the following individuals: I would like to thank my thesis advisor Professor Victoria Orphan. She has opened up a world of possibilities for me since joining her lab. I am very grateful to have the rare opportunity and freedom to learn the best techniques and explore my own ideas in science in the past six years, while knowing that she will always be there to support and guide regardless of the situation. She has helped to build a solid foundation for me to pursue whichever career path I decide on next. I would also like to thank Professor Jared Leadbetter. He is my thesis advisory committee chair and a living encyclopedia of microbiology. I have been fortunate to interact and learn from him since my first day at Caltech. Not only have I been fortunate to perform research in his laboratory, but I have also learned a lot by being a teaching assistant in his class for three consecutive years and chatting with him about microbial physiology and microbiology in general. Professors Dianne Newman and Woodward Fischer have also provided excellent guidance as members in my thesis advisory committee. Their extremely approachable personalities combined with their immense knowledge in fields of microbial metabolism, microbial interactions, and geobiology helped to improve this thesis from the small details to the big picture.