The Photon Identi Cation Loophole in EPRB Experiments
Total Page:16
File Type:pdf, Size:1020Kb
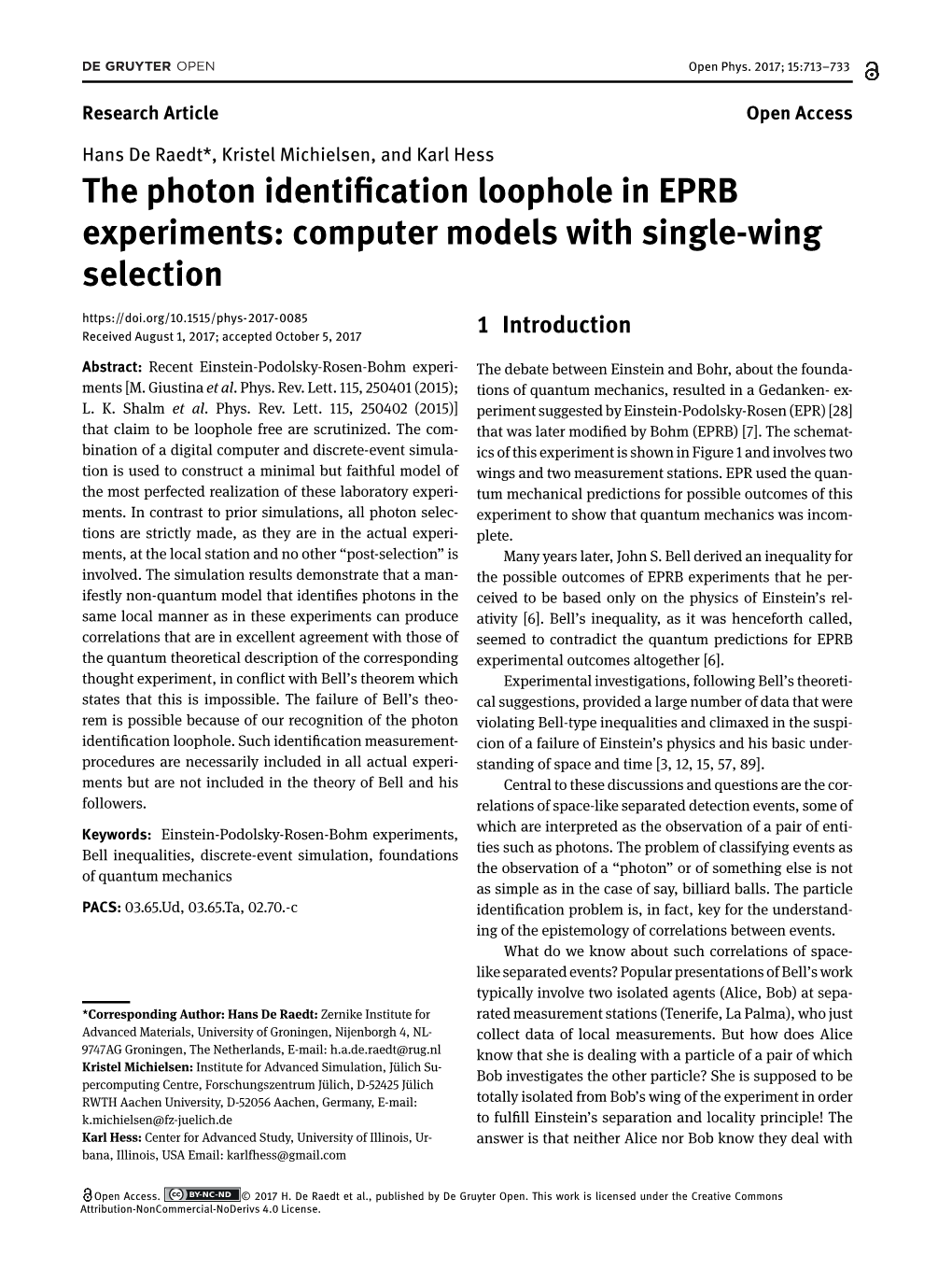
Load more
Recommended publications
-
Choquette Seminar.Ai
NOIS AT U LI RB IL A F N O A - Y C T H I A S M CENTER FOR NANOSCALE SCIENCE AND TECHNOLOGY R P E A V I I G .............................................................................................................. N N U University of Illinois at Urbana-Champaign CENTER FOR NANOSCALE CHEMICAL-ELECTRICAL- MECHANICAL MANUFACTURING SYSTEMS Nanotechnology: Feynman's "Room at the Bottom" and the Tyranny of Large Systems Richard Feynman's famous essay "There is plenty of room at the bottom" has initiated much interest in nano-science research and many of Feynman's dreams have become reality as semiconductor devices approach atomistic length-scales. It has become clear, however, that any room gained at the "bottom" brings ever growing necessities for the large systems that are felt by design engineers as smothering tyranny. A well known example is the heat generation of VLSI systems. Any resolution of these problems is connected to enormous costs for the chip industry and is therefore only available for the conventional technology and not for new and risky ideas. The problems of scaling novel devices for conventional systems are therefore insurmountable at universities and smaller research institutions and it is natural to look for new and revolutionary systems in connection with new and revolutionary devices. In this lecture, I will discuss two proposals for new types of system design: (i) systems that work in analogy to the biological systems of nature and (ii) systems based on the principles of quantum mechanics e.g. the Quantum Computer. The only working giant systems containing nano-devices are the actual objects of biology. -
About the Beckman Institute
Annual Report 2010-2011 FBOR eADcVAkNCmED SaCInENCE IAnND sTtEiCHtNuOLtOeGY ABOUT THE BECKMAN INSTITUTE he Beckman Institute for Advanced The Beckman Institute is also home to The 313,000-square-foot building was Science and Technology at the three strategic initiatives that seek to made possible by a generous gift from TUniversity of Illinois at Urbana- unify campus activities in their respective University of Illinois alumnus and founder Champaign is an interdisciplinary areas: of Beckman Instruments, Inc., Arnold research institute devoted to leading- • HABITS O. Beckman, and his wife Mabel M. edge research in the physical sciences, • Imaging Beckman, with a supplement from computation, engineering, biology, • Social Dimensions of Environmental the State of Illinois. behavior, cognition, and neuroscience. Policy The Institute’s primary mission is to foster Additionally, the Arnold and Mabel interdisciplinary work of the highest qual - More than 1,000 researchers from more Beckman Foundation provides ongoing ity, transcending many of the limitations than 40 University of Illinois departments financial assistance for various Institute inherent in traditional university organi - as diverse as psychology, computer and campus programs. Daily operating zations and structures. The Institute was science, electrical and computer engi - expenses of the Institute are covered by founded on the premise that reducing neering, and biochemistry, comprising the state and its research programs are the barriers between traditional scientific 14 Beckman Institute groups, work within mainly supported by external funding and technological disciplines can yield and across these overlapping areas. The from the federal government, corpora - research advances that more conven - building offers more than 200 offices; tions, and foundations. -
PNP What Is in a Name?
PNP What is in a Name? Robert S. Eisenberg Department of Applied Mathematics Illinois Institute of Technology Department of Physiology and Biophysics Rush University Medical Center Chicago IL [email protected] July 25, 2019 File: PNP what is in a name July 25-1 2019.docx 1 Abstract and Summary The name PNP was introduced by Eisenberg and Chen because it has important physical meaning beyond being the first letters of Poisson-Nernst-Planck. PNP also means Positive-Negative-Positive, the signs of majority current carriers in different regions of a PNP bipolar transistor. PNP transistors are two diodes in series PN + NP that rectify by changing the shape of the electric field. Transistors can function as quite different types of nonlinear devices by changing the shape of the electric field. Those realities motivated Eisenberg and Chen to introduce the name PNP. The pun “PNP = Poisson-Nernst-Planck = Positive-Negative-Positive” has physical content. It suggests that Poisson-Nernst-Planck systems like open ionic channels should not be assumed to have constant electric fields. The electric field should be studied and computed because its change of shape is likely to be important in the function of biological systems, as it is in semiconductor systems. 2 PNP is a shortened name for “Positive-Negative-Positive” or “Poisson-Nernst- Planck equation”. It was not meant to be just an abbreviation: names are important, beyond their logical meaning, as the world shows us everyday. The name PNP is no exception. The name was chosen to help understand the system it describes. PNP was a pun introduced at the 1993 Biophysical Society (USA) meeting [1] by Eisenberg and Chen [2, 3] to emphasize the analogy between open ion channels and semiconductor devices. -
Annual Report
Annual Report BECKMAN INSTITUTE FOR ADVANCED SCIENCE AND TECHNOLOGY 2 0 0 0 UNIVERSITY OF ILLINOIS AT URBANA-CHAMPAIGN BIHighlights in 2000 ❏ How do you isolate, characterize, and manipu- TABLE OF CONTENTS late molecules in amounts so small that Mother Nature created special containers inside single cells 2000 MESSAGE FROM THE DIRECTOR just to transport them? In 2000, Beckman Institute Jiri Jonas, Director pages 2–4 faculty members Paul Bohn, Mark Shannon, and MOLECULAR AND ELECTRONIC NANOSTRUCTURES Jonathan Sweedler initiated a new collaborative pro- Overview pages 6–7 ject funded through the Defense Advanced Research Projects Agency to design a new Karl Hess and Jeffrey S. Moore, Co-chairs category of measurement device, the Biofluidic Intelligent Processor. This device will Highlights pages 8–12 allow the active manipulation, detection, and characterization of biological fluids with BIOLOGICAL INTELLIGENCE volumes more than a million times smaller than a single drop of blood. At the heart of Overview pages 14–15 the Biofluidic Intelligent Processor are molecular gates with active areas not much Gary S. Dell and William T. Greenough, Co-chairs larger than transistors in microprocessors. The molecular gates can intelligently sepa- Highlights pages 16–20 rate specific components, passing the rest, concentrating them in attoliter volumes, HUMAN-COMPUTER INTELLIGENT INTERACTION then digitally tagging them for detection. As digitizing electronics has permitted com- Overview pages 22–23 Thomas S. Huang and Arthur F. Kramer, Co-chairs plex operations to be processed, digitizing molecular fluid flow can potentially solve the Highlights pages 24–28 daunting challenges posed by trace levels of extraordinarily lethal toxins. -
Monte Carlo Device Simulation Full Band and Beyond Series: the Springer International Series in Engineering and Computer Science
springer.com Karl Hess (Ed.) Monte Carlo Device Simulation Full Band and Beyond Series: The Springer International Series in Engineering and Computer Science Monte Carlo simulation is now a well established method for studying semiconductor devices and is particularly well suited to highlighting physical mechanisms and exploring material properties. Not surprisingly, the more completely the material properties are built into the simulation, up to and including the use of a full band structure, the more powerful is the method. Indeed, it is now becoming increasingly clear that phenomena such as reliabil• ity related hot-electron effects in MOSFETs cannot be understood satisfac• torily without using full band Monte Carlo. The IBM simulator DAMOCLES, therefore, represents a landmark of great significance. DAMOCLES sums up the total of Monte Carlo device modeling experience of the past, and reaches with its capabilities and opportunities into the distant future. This book, 1991, X, 310 p. therefore, begins with a description of the IBM simulator. The second chapter gives an advanced introduction to the physical basis for Monte Carlo simulations and an outlook on why Printed book complex effects such as collisional broadening and intracollisional field effects can be Hardcover important and how they can be included in the simulations. References to more basic intro• 199,99 € | £179.99 | $249.99 the book. The third chapter ductory material can be found throughout describes a typical [1]213,99 € (D) | 219,99 € (A) | CHF relationship of Monte Carlo simulations to experimental data and indicates a major difficulty, 236,00 the vast number of deformation poten• tials required to simulate transport throughout the Softcover entire Brillouin zone. -
1 Curriculum Vitae Leonard Franklin Register II
Curriculum Vitae Leonard Franklin Register II TITLE: Associate Professor DEPARTMENT: Electrical and Computer Engineering (and Microelectronics Research Center) UNIVERSITY: The University of Texas at Austin EDUCATION: North Carolina State University Electrical Engineering B.S. (summa cum Laude) Spring 1983 North Carolina State University Physics B.S. (summa cum Laude) Spring 1983 North Carolina State University Elec. & Comp. Eng. Ph.D.1 Spring 19902 ACADEMIC POSITIONS: The University of Texas at Austin Temple Foundation Faculty Fellow, #4 Fall 2006‐present The University of Texas at Austin Associate Professor Fall 2005‐present The University of Texas at Austin Assistant Professor Spring 2000‐Summer 2005 University of Illinois at U‐C Research Scientist Spring 2003‐Fall 1999 University of Illinois at U‐C Visiting Research Assistant Prof. Spring 2000‐Fall 1992 VITA Leonard Franklin Register is an Associate Professor of Electrical and Computer Engineering at the University of Texas at Austin, a member of the Microelectronics Research Center, and recipient of the Temple Foundation Endowed Faculty Fellowship #4. He has B. S. degrees both in Electrical Engineering and in Physics, and a Ph. D. in Electrical and Computer Engineering, each from North Carolina State University. He was a research scientist in the Computational Electronics group within the Beckman Institute at the University of Illinois at Urbana‐Champaign before joining the faculty of The University of Texas at Austin in 2000. Prof. Register is a device theorist whose research is focused on understanding and modeling the essential physics underlying the operation of nanoelectronic and optoelectronic devices. His current research interests included alternative materials and device geometries for CMOS (non‐classical CMOS), alternative switching methods and/or state variables (beyond CMOS), quantum‐ corrected semiclassical transport, and quantum transport with scattering. -
Enhancing Support of Transformative Research at the National Science Foundation
NSB-07-32 Enhancing Support of Transformative Research at the National Science Foundation May 7, 2007 National Science Board Steven C. Beering, Chairman, President Emeritus, Purdue University, West Lafayette Kathryn D. Sullivan, Vice Chairman, Director, Battelle Center for Mathematics and Science Education Policy, John Glenn School of Public Affairs, Ohio State University, Columbus Mark R. Abbott, Dean and Professor, College of Oceanic and Atmospheric Sciences, Oregon State University Dan E. Arvizu, Director and Chief Executive, National Renewable Energy Laboratory, Golden, Colorado Barry C. Barish, Maxine and Ronald Linde Professor of Physics Emeritus and Director, LIGO Laboratory, California Institute of Technology Camilla P. Benbow, Patricia and Rodes Hart Dean of Education and Human Development, Peabody College, Vanderbilt University Ray M. Bowen, President Emeritus, Texas A&M University, College Station John T. Bruer, President, The James S. McDonnell Foundation, St. Louis G. Wayne Clough, President, Georgia Institute of Technology Kelvin K. Droegemeier, Associate Vice President for Research, Regents’ Professor of Meteorology and Weathernews Chair, University of Oklahoma, Norman Kenneth M. Ford, Director and Chief Executive Officer, Institute for Human and Machine Cognition, Pensacola Patricia D. Galloway, Chief Executive Officer, The Nielsen-Wurster Group, Inc., Seattle José-Marie Griffiths, Dean, School of Information and Library Science, University of North Carolina, Chapel Hill Daniel E. Hastings, Dean for Undergraduate Education and Professor, Aeronautics & Astronautics and Engineering Systems, Massachusetts Institute of Technology Karl Hess, Professor of Advanced Study Emeritus and Swanlund Chair, University of Illinois, Urbana-Champaign Elizabeth Hoffman, Executive Vice President and Provost, Iowa State University, Ames Louis J. Lanzerotti, Distinguished Research Professor of Physics, Center for Solar-Terrestrial Research, New Jersey Institute of Technology Alan I. -
Advanced Theory of Semiconductor Devices Karl Hess
To purchase this product, please visit https://www.wiley.com/en-us/9780780334793 Advanced Theory of Semiconductor Devices Karl Hess Hardcover 978-0-780-33479-3 December 1999 Print-on- $198.75 demand DESCRIPTION Semiconductor devices are ubiquitous in today's world and found increasingly in cars, kitchens, and electronic door looks, attesting to their presence in our daily lives. This comprehensive book brings you the fundamentals of semiconductor device theory from basic quantum physics to computer aided design. Advanced Theory of Semiconductor Devices will help improve your understanding of computer simulation devices through a thorough discussion of basic equations, their validity, and numerical solutions as they are contained in current simulation tools. You will gain state-of-the-art knowledge of devices used in both III-V compounds and silicon technology. Specially featured are novel approaches and explanations of electronic transport, particularly in p-n junction diodes. Close attention is also given to innovative treatments of quantum level laser diodes and hot electron effects in silicon technology. This in-depth book is designed expressly for graduate students, research scientists, and research engineers in solid state electronics who want to gain a better grasp of the principles underlying semiconductor devices. ABOUT THE AUTHOR About the Author Karl Hess holds the Swanlund Endowed Chair and is professor of electrical and computer engineering and of physics at the University of Illinois, Urbana-Champaign. Dr. Hess has dedicated a major portion of his research to electronic transport in semiconductors and semiconductor devices, with particular emphasis on hot electron effects and effects pertinent to device miniaturization. -
Biography of Karl Hess BIOGRAPHY
Biography of Karl Hess BIOGRAPHY hysicists have strived for centu- back,’’ Hess said. His persistence paid ries to understand the behavior off; in 1977, the University of Illinois of electrons, information crucial offered him a visiting associate profes- P for explaining electricity and the sorship. physical properties of all materials. Al- though electrons are exponentially too Supercomputers, Super Simulations small to see, researchers have developed After Hess returned to Illinois, he a variety of techniques for simulating worked to improve the efficiency of electronic nature and movement with charge-coupled devices, semiconductor computer models. A leader in this field chips that record images in video cam- is Karl Hess, professor of electrical and eras. However, craving more basic study, computer engineering at the University he soon teamed up with engineering of Illinois, Urbana. professor Ben Streetman, now at the With his colleagues, Hess has devel- University of Texas at Austin, to investi- oped numerous simulation tools for dis- gate a broader class of semiconductor cerning the behavior of electrons in materials and devices. The two scientists solids, semiconductor lasers, and other developed the concept of ‘‘real space devices. His work has earned him many Karl Hess. transfer’’ (4), which explains the perfor- grants and accolades, including the rare mance of certain high-frequency transis- dual election to both the National Acad- fessor at the University of Vienna. A tors. Streetman also mentored Hess on emy of Engineering (2001) and the Na- the politics of American university life. tional Academy of Sciences (2003). In year later, he met University of Illinois professor John Bardeen, who was on a His advice helped Hess land a tenured his Inaugural Article (1), published in professorship of electrical and computer lecture tour throughout Europe. -
Closing the Door on Quantum Nonlocality
entropy Article Closing the Door on Quantum Nonlocality Marian Kupczynski Département d’informatique et d’ingénierie, Université du Québec en Outaouais, Case postale 1250, succursale Hull, Gatineau, QC J8X 3X7, Canada; [email protected]; Tel.: +1-819-777-4493 Received: 11 September 2018; Accepted: 8 November 2018; Published: 15 November 2018 Abstract: Bell-type inequalities are proven using oversimplified probabilistic models and/or counterfactual definiteness (CFD). If setting-dependent variables describing measuring instruments are correctly introduced, none of these inequalities may be proven. In spite of this, a belief in a mysterious quantum nonlocality is not fading. Computer simulations of Bell tests allow people to study the different ways in which the experimental data might have been created. They also allow for the generation of various counterfactual experiments’ outcomes, such as repeated or simultaneous measurements performed in different settings on the same “photon-pair”, and so forth. They allow for the reinforcing or relaxing of CFD compliance and/or for studying the impact of various “photon identification procedures”, mimicking those used in real experiments. Data samples consistent with quantum predictions may be generated by using a specific setting-dependent identification procedure. It reflects the active role of instruments during the measurement process. Each of the setting-dependent data samples are consistent with specific setting-dependent probabilistic models which may not be deduced using non-contextual local realistic or stochastic hidden variables. In this paper, we will be discussing the results of these simulations. Since the data samples are generated in a locally causal way, these simulations provide additional strong arguments for closing the door on quantum nonlocality. -
Slava (Vyacheslav) V. Rotkin
Curriculum Vitae Slava (Vyacheslav) V. Rotkin 16 Memorial Drive East, Bethlehem, PA 18015 Physics Department, Lehigh University, voice/phone: (610) 758-3904 fax: (610) 758-5730 e-mail: [email protected] ; web page http://theory.physics.lehigh.edu/rotkin Education: 4/94 - 4/97 Ph.D. Ioffe Institute, St. Petersburg, Russia. Advisor Prof. Robert A. Suris. Ph.D. degree in physics and mathematics received from Ioffe Institute. Thesis "Modeling of the electronic structure, formation and interaction processes in nanoscale carbon-based clusters". 2/96 - 6/96 Course on the Modern Statistical Physics: Random Systems, University of Oslo, Norway. 9/90 - 3/93 Extramural Language courses, St. Petersburg Electro-technical University, Russia. 9/90 - 12/93 Extramural courses taken at Ioffe Institute, St. Petersburg, "Band structure of semiconductors". 9/90 - 12/93 Extramural courses taken at St. Petersburg Technical University "Optics of semiconductors", "Group theory application in condensed matter", "Condensed Matter Physics". 8/86 - 2/94 M.Sc. (Honors) Electro-technical University, St. Petersburg, Russia. Major: Optoelectronics, Electronics. Graduated with Honors. Thesis "C60 molecule spherical shell quantum well model". Advisor Prof. Robert A. Suris. Research experience (12 years): 8/04 - present Assistant Professor, Physics Department, Lehigh University, Bethlehem PA, USA. 3/03 - 8/04 Visiting Research Assistant Professor, Beckman Institute, UIUC, Urbana IL, USA. 3/00 - 3/03 Beckman Fellow (Postdoctoral Research Associate), Beckman Institute, UIUC, Urbana IL, USA. 12/99 - 3/00 Visiting Research Associate, Beckman Institute, UIUC, Urbana IL, USA. 2/99 - 8/04 Research Staff Fellow, Laboratory of Theoretical Bases of Microelectronics of Solid State Electronics Division of Ioffe Physico-technical Institute, St. -
Manuel Aschwanden, ``A Classical View of Quantum Entanglement
Winter Semester 2004/2005 Prof. Dr. K. Hess Master’s Thesis at the Department of Information Technology and Electrical Engineering A classical view of quantum entanglement by Manuel Aschwanden UIUC Supervisor: Prof. Dr. K. Hess ETHZ Advisor: Prof. Dr. W. Fichtner ETHZ Co-Advisor: Prof. Dr. A. Schenk Issue Date: October 4, 2004 Submission Date: April 3, 2005 II Acknowledgments First and most of all, I would like to thank Prof. Karl Hess for giving me the op- portunity to work with him on the challenging topic of quantum information. I have had the benefit of many discussions with Professor Hess on quantum mechanics, in- formation theory and related areas in physics. I would also like to thank Professor Walter Philipp for introducing me to Kolmogorov’s probability theory and Salvador Barraza-Lopes for helpful discussions and commentary. I also wish to express my gratitude to my two supervisors, Professor Wolfgang Fichtner and Professor Andreas Schenk, at the Integrated Systems Laboratory at ETH Zurich. Without the help of Professor Fichtner and the financial support of IIS, this work would not have been possible. Urbana, April 3, 2005 Manuel Aschwanden III IV Abstract This Master’s Thesis addresses the question of what is quantum entanglement. This question is of importance for engineering and information theory because of the pos- sibility of quantum computing. Key to the answer of this question is the debate between Einstein and Bohr relating to this subject. Throughout this thesis the view of Einstein is given special and only consideration. It is appropriate to examine this view 100 years after Einstein’s famous papers that have laid the foundation and given the principles for this debate.