The Vortex Lattice of a Type II Superconductors Studied by Small Angle Neutron Scattering
Total Page:16
File Type:pdf, Size:1020Kb
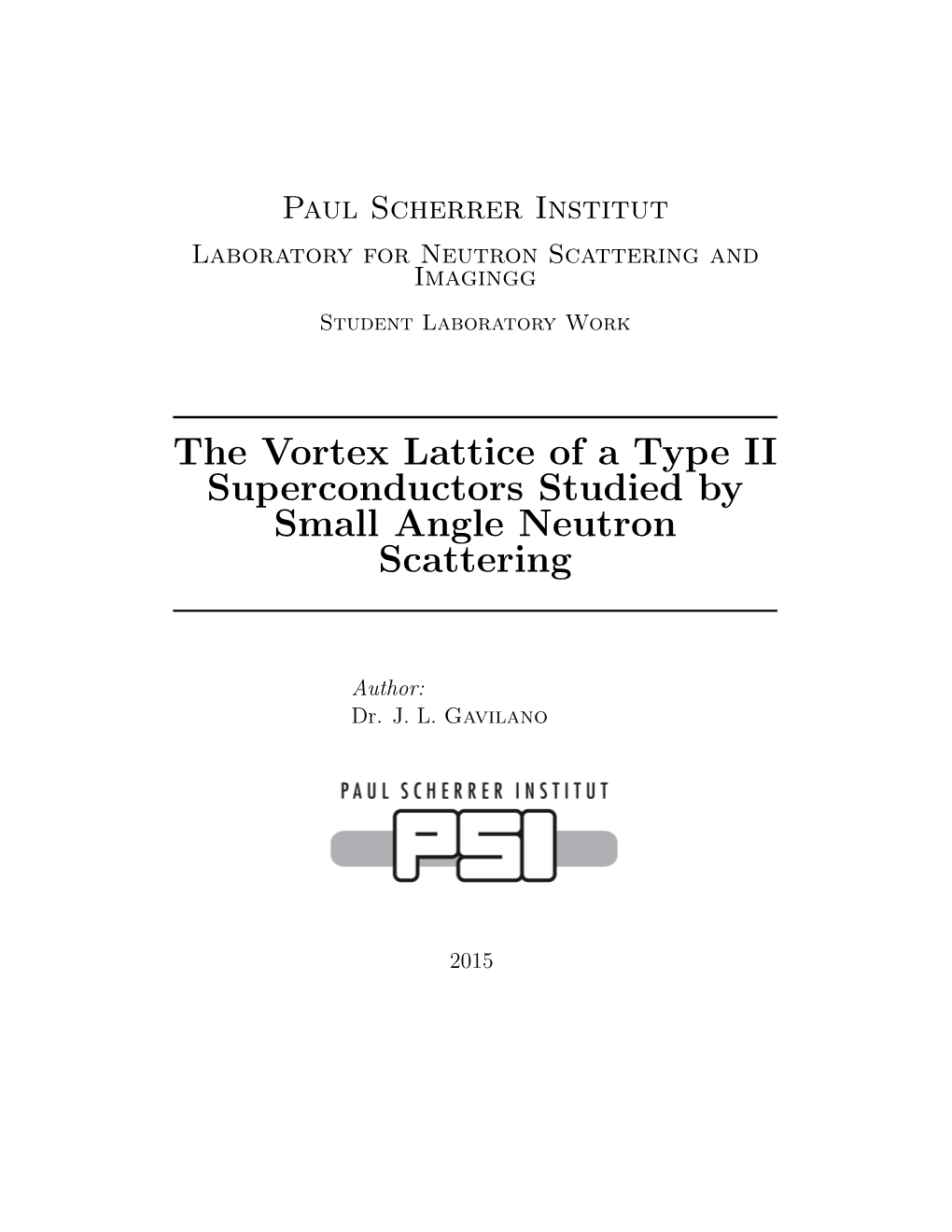
Load more
Recommended publications
-
A Search for Free Oscillations at the ESS N
A search for free n → n oscillations at t he ESS π π ? n n π π π D. Milstead Stockholm University Why baryon number violation ? Why baryon number violation ? • Baryon number is not a ”sacred” quantum number – Approximate conservation of BN in SM • ”Accidental” global symmetry at perturbative level – Depends on specific matter content of the SM • BNV in SM by non-perturbative processes –Sphalerons – B-L conserved in SM, not B,L separately. – Generic BNV in BSM theories, eg, SUSY. – BNV a Sakharov condition for baryogenesis Why n→ n ? n→ n • Theory • Baryogenesis via BNV (Sakharov condition) • SM extensions from TeV mass scales scale-upwards • Complementarity with open questions in neutrino physics • Experiment • One of the few means of looking for pure BNV • Stringent limit on stability of matter Neutron oscillations – models • Back-of-envelope dimensional reasoning: cΛ6 6 q operator for ∆B =2, ∆ L = 0⇒ δ m= QCD ⇒ M ∼ 1000 TeV n→ n M 5 • R-parity violating supersymmetry • Unification models: M ∼ 1015 GeV • Extra dimensions models • Post-sp haleron baryogenesis • etc, etc: []arXiv:1410.1100 High precision n→ n search ⇒ Scan over wide range of phase space for generic BNV + ⇒ model constai nts. Extend sensitivity in RPV-SUSY ATLAS multijet ATLAS CMS dijet CMS ESS Arxiv:1602.04821 (hep-ph) Displaced jets RPV-SUSY – TeV-scale sensitivity Neutrino physics ⇔ neutron oscillations Neutrinoless 2β -decay n→ n Eg seesaw mechanism for light ν Eg Unification models ∆L =2, ∆ B = 0, ∆L =0, ∆ B = 2, 2 ∆()B − L = 2 ∆()B − L = Neutrinoless 2β -decay ⇔ nn → linked under BL - viol ation. -
ICANS XXI Dawn of High Power Neutron Sources and Science Applications
Book of Abstracts ICANS XXI Dawn of high power neutron sources and science applications 29 Sep - 3 Oct 2014, JAPAN Ibaraki Prefectural Culture Center Update : 12 Oct. 2014 Best photography in 7th Oarai Town Photo Contest. WELCOME TO ICANS XXI ICANS (International Collaboration on Advanced Neutron Sources) is a network for scientists who are involved in developing pulsed neutron sources and accelerator based spallation neutron sources. Since 1st ICANS meetings was held in 1977 at Argonne National Laboratory in the day of dawn of spallation neutron technique, ICANS has been continuously held already 20 times somewhere in the world. Now we are extremely happy to announce that the ICANS, the 21st meeting, will be held at Mito hosted by J-PARC this autumn. We have a large number of topics to be discussed, there are twelve topics, such as futuristic idea of neutron source, rapid progress in facilities, integration issues in target-moderator-development, etc. The details can be found in the agenda. The meeting has a two layered structure, one is plenary session and another is workshop. Two of them are complementary and tightly cooperate each other. In the meeting we would like to enhance "workshop style", which is an original and traditional way of ICANS. Actually 2/3 of topics will be discussed in the workshop sessions. It also will be essentially organized/ lead by the workshop chairs. Plenary session shows overall issues in a relevant workshop, whose details should be talked/discussed in the workshop. The venue for the meeting is Mito city, where the 2nd Shogun Family lived for a long period of time during Edo era from 17th to 19th century, when the Tokugawa shogunate ruled the country. -
The HIBEAM Experiment for the European Spallation Source
The HIBEAM Experiment for the European Spallation Source n ? n D. Milstead Stockholm University Outline 1. The aims of the experiment 2. The physics case 3. The proposed program at the ESS 4. Status HIBEAM High Intensity Baryon Extraction and Measurement Search for • 푛 → 푛ത • 푛 → 푛′ (mirror neutrons) Also measurements of weak nucleon-nucleon interactions. Baryon and lepton number violation • BN,LN ”accidental” SM symmetries at perturbative level – BNV, LNV in SM non-perturbatively (eg instantons) – B-L is conserved, not B, L separately. • BNV, LNV needed for baryogenesis and leptogenesis • BNV,LNV generic features of SM extensions (eg SUSY) nn Dimensional reasoning: c6 6q operator for B 2, L 0 m QCD M 1000 TeV nn M 5 R-parity violating supersymmetry Unification theories: M 1015 GeV Extra dimensions models Post-sphaleron baryogenesis etc, etc: arXiv:1410.1100 High precision n n search Scan over wide range of phase space for generic BNV + model constaints. RPV-SUSY Super-K ILL LHC, flavour Constraints vanish for >> TeV masses nnbar@ESS: extends mass range by up to ~400 TeV cf Super-K : pushes into the PeV scale Complementary B,L-violation observables BL 1, BL 2, 0, BL 0, 2, BL 0 BL 2 BL 2 Symbiosis n n , NN decay. Nucleon decay Stable proton. 0 Few pure BNV searches Neutron oscillations – an experimentalist’s view Hypothesis: baryon number is weakly violated. How do we look for BNV? Single nucleon decay searches, eg, pe 0 ? L-violation, another (likely weakly) violated quantity. Decays without leptons, eg, p , impossible due to angular momentum conservation. -
Instrumental Aspects
EPJ Web of Conferences 155, 00002 (2017) DOI: 10.1051/epjconf/201715500002 JDN 22 Instrumental aspects Navid Qureshi Institut Laue Langevin, 38000 Grenoble, France Abstract. Every neutron scattering experiment requires the choice of a suited neutron diffractometer (or spectrometer in the case of inelastic scattering) with its optimal configuration in order to accomplish the experimental tasks in the most successful way. Most generally, the compromise between the incident neutron flux and the instrumental resolution has to be considered, which is depending on a number of optical devices which are positioned in the neutron beam path. In this chapter the basic instrumental principles of neutron diffraction will be explained. Examples of different types of experiments and their respective expectable results will be shown. Furthermore, the production and use of polarized neutrons will be stressed. 1. Introduction A successful neutron scattering experiment requires a thorough plan of which instrument to use and how to set up its configuration in order to obtain the best possible results. Optimally, the decision of the set-up is taken beforehand, but in many cases the instrument parameters are modified on-the-fly after analyzing the first results. Due to the different experimental tasks – reaching from magnetic or nuclear structure investigation to the mapping of phase diagrams – and the different sample dimensions (both the crystal size and the lattice parameters), which can be investigated at one and the same instrument, it becomes obvious that the versatility of some instruments has to be adjusted to satisfy the experimental tasks. Obviously, the sample state (powder, single crystal, liquid, etc.) limits the choice of instruments, however, you can find powder diffractometers which differ from each other concerning the used neutron wavelength and particular devices in the beam path in order to maximize the efficiency for a type of experiment. -
Viii-I. Summary of Research Activities
VIII-I. SUMMARY OF RESEARCH ACTIVITIES VIII-I-1. MEETINGS AND SEMINARS Specialists’ Meetings Held in the FY 2013 1. Issues in Radiogenic Circulatory Disease and Cataracts 2. Proceedings of Workshop on Reactor Physics 3. The Results and Future Prospects of Activation Analysis Using KUR" 4. Workshop on Materials Irradiation Effects and Applications 5. Proceedings of the Specialist Research Meeting on Science and Engineering of Unstable Nuclei and Their Uses on Condensed Matter Physics III 6. Proceedings of the Specialist Research Meeting on “Abnormal Protein Aggregation and the Folding Diseases, and their Protection and Repair System” (VI) 7. Proceedings of the Symposium on Present and Future Statuses of Criticality Safety Research 8. Novel Development of BNCT - From Special to General - 9. Proceedings of the Specialists' Meeting on the Chemistry and Technology of Actinide Elements Proceedings of the Specialist Research Meeting on Science and Engineering of Unstable Nuclei and Their 10. Technological Development, Operation, and Data Analysis of Radiation Mapping Systems in Affected Area of Nuclear Accident 11. Proceedings of the Specialist Meeting on Positron Annihilation Study for Science and Engineering 2013 12. Proceedings of the Specialists' Meeting on Radioactive Wastes Management 13. Neutron Imaging Workshops Organized in the FY 2013 1. Promotion of Leading Research toward Effective Utilization of Multidisciplinary Nuclear Science and Technology 2. Workshop of Next Neutron Source for Beam Utilization after KUR II Special Meeting Held in the FY2013 Meeting on the Future Project of the Kyoto University Research Reactor Institute VIII-I-2. COLLABORATION RESEARCH AND VISITING SCIENTISTS Visiting Scientists The number of project researches .................................................. 11 (The number of allotted research subject) ............................... -
Hunting the Missing Baryon Number Violation at the ESS
Hunting the missing baryon number violation at the ESS n ? n D. Milstead Stockholm University Outline • Why look for neutron oscillations ? • How to look for neutron oscillations • Nnbar and HIBEAM at the ESS Baryon and lepton number violation • BN,LN ”accidental” SM symmetries at perturbative level – BNV, LNV in SM non-perturbatively (eg instantons) – B-L is conserved, not B, L separately. • BNV, LNV needed for baryogenesis and leptogenesis • BNV,LNV generic features of SM extensions (eg SUSY) • Need to explore the possible selection rules: BLBL 0 , 0, 0 BLBL 0 , 0, 0 LBBL 0 , 0, 0 ..... Neutron oscillation Mirror neutrons Neutron-antineutron oscillations R-parity violating supersymmetry, minimal flavour violation SUSY Unification models: M 1015 GeV Left-right symmetric models (nn and 0 2 ) Extra dimensions models Post-sphaleron baryogenesis etc, etc: arXiv:1410.1100 High precision n n search Scan over wide range of phase space for generic BNV + model constaints. Operator analysis Six quark operators i : n n, NN NN KK 1 Eg : n n neff n5 i n i n M X i Short distance (RPV SUSY): i 6 Long distance Hadronic ME: nni QCD 1 M 5 Oscillation time = X 6 nneff QCD Beyond the TeV scale Super-K ILL LHC, flavour Constraints vanish for >> TeV masses nnbar@ESS: extends mass range by up to ~400 TeV cf Super-K : pushes into the PeV scale : Reach beyond the LHC An experimentalist’s view (1) Hypothesis: baryon number is weakly violated. How do we look for it ? Need processes in which only BNV takes place. Single nucleon decay searches, eg, pe 0 ? BL 11 , ! Decays without leptons, eg, p , impossible due to angular momentum conservation. -
Program—Advances in Neutron Facilities, Instrumentation & Software
As of July 10, 2020 American Conference on Neutron Scattering Advances in Neutron Facilities, Instrumentation and Software * Invited Paper SESSION A02.01: Neutron Source become the leading institution in neutron science in Latin America. Main challenges of the LAHN project and Facilities I are (i) to implement a suite of state-of-the-art neutron scattering instruments, (ii) to build a solid neutron scattering user community, (iii) to form the human A02.01.01* resources necessary for defining, implementing, Neutron Scattering Facilities at the McMaster operating and using the instruments. Nuclear Reactor In this work we present the strategies and activities Patrick Clancy; McMaster University, Canada developed to achieve these goals, and the current state of the project. This includes a description of the The McMaster Nuclear Reactor (MNR) is a 5 MW suite of eight instruments defined for the first stage of open-pool reactor located on the campus of the laboratory, together with their scientific cases and McMaster University in Hamilton, Ontario. the different strategies adopted for their provision. Following the closure of the NRU Reactor at Chalk We also describe a comprehensive program River in March 2018, MNR is the only facility in developed to foster the user community, train LAHN Canada with neutron scattering capabilities. At human resources and increase LAHN visibility in present, MNR is home to two neutron scattering order to reach a wider group of stakeholders. instruments: the McMaster Alignment Diffractometer (MAD), a general purpose triple-axis spectrometer, A02.01.03 and the McMaster Small Angle Neutron Scattering Neutron Scattering at Missouri—Current Status beamline (MacSANS). -
From X-Ray Telescopes to Neutron Scattering: Using Axisymmetric Mirrors to Focus a Neutron Beam
From x-ray telescopes to neutron scattering: Using axisymmetric mirrors to focus a neutron beam The MIT Faculty has made this article openly available. Please share how this access benefits you. Your story matters. Citation Khaykovich, B. et al. “From x-ray telescopes to neutron scattering: Using axisymmetric mirrors to focus a neutron beam.” Nuclear Instruments and Methods in Physics Research Section A: Accelerators, Spectrometers, Detectors and Associated Equipment 631.1 (2011): 98-104. As Published http://dx.doi.org/10.1016/j.nima.2010.11.110 Publisher Elsevier Version Author's final manuscript Citable link http://hdl.handle.net/1721.1/61704 Terms of Use Article is made available in accordance with the publisher's policy and may be subject to US copyright law. Please refer to the publisher's site for terms of use. Published: B. Khaykovich, et al., Nucl. Instr. and Meth. A (2010), doi:10.1016/j.nima.2010.11.110 From x-ray telescopes to neutron scattering: using axisymmetric mirrors to focus a neutron beam B. Khaykovich1*, M. V. Gubarev2, Y. Bagdasarova3, B. D. Ramsey2, and D. E. Moncton1,3 1Nuclear Reactor Laboratory, Massachusetts Institute of Technology, 138 Albany St., Cambridge, MA 02139, USA 2Marshall Space Flight Center, NASA, VP62, Huntsville, AL 35812, USA 3Department of Physics, Massachusetts Institute of Technology, 77 Massachusetts Ave., Cambridge, MA 02139, USA Abstract We demonstrate neutron beam focusing by axisymmetric mirror systems based on a pair of mirrors consisting of a confocal ellipsoid and hyperboloid. Such a system, known as a Wolter mirror configuration, is commonly used in x-ray telescopes. -
2.4 Neutron Diffraction
Open Research Online The Open University’s repository of research publications and other research outputs Combined Neutron Imaging and Diffraction: Instrumentation and Experimentation Thesis How to cite: Burca, Genoveva (2013). Combined Neutron Imaging and Diffraction: Instrumentation and Experimentation. PhD thesis The Open University. For guidance on citations see FAQs. c 2013 The Author https://creativecommons.org/licenses/by-nc-nd/4.0/ Version: Version of Record Link(s) to article on publisher’s website: http://dx.doi.org/doi:10.21954/ou.ro.0000f116 Copyright and Moral Rights for the articles on this site are retained by the individual authors and/or other copyright owners. For more information on Open Research Online’s data policy on reuse of materials please consult the policies page. oro.open.ac.uk crrei> Combined Neutron Imaging and Diffraction: Instrumentation and Experimentation Thesis submitted to The Open University for the degree of Doctor of Philosophy in the Faculty of Mathematics, Computing and Technology (MCT) By Genoveva Burca Materials Engineering Group The Open University September 2012 ProQuest Number: 13835948 All rights reserved INFORMATION TO ALL USERS The quality of this reproduction is dependent upon the quality of the copy submitted. In the unlikely event that the author did not send a com plete manuscript and there are missing pages, these will be noted. Also, if material had to be removed, a note will indicate the deletion. uest ProQuest 13835948 Published by ProQuest LLC(2019). Copyright of the Dissertation is held by the Author. All rights reserved. This work is protected against unauthorized copying under Title 17, United States C ode Microform Edition © ProQuest LLC. -
Self-Shielding Copper Substrate Neutron Supermirror Guides
Self-Shielding Copper Substrate Neutron Supermirror Guides P. M. Bentley1,5, R. Hall-Wilton1,3,4, C. P. Cooper-Jensen1, N. Cherkashyna1, K. Kanaki1, C. Schanzer2, M. Schneider2, P. B¨oni2 E-mail: [email protected], [email protected], [email protected], [email protected], [email protected], [email protected], [email protected], [email protected] 1 European Spallation Source ERIC, Box 176, 221 00 Lund, Sweden 2 Swiss Neutronics AG Neutron Optical Components & Instruments, Br¨uhlstrasse 28, CH-5313 Klingnau, Switzerland 3 Universit`adegli Studi di Milano-Bicocca, Piazza della Scienza 3, 20126 Milano, Italy 4 University of Glasgow, Glasgow, United Kingdom 5 Anderson Butovich Ltd. United Kingdom Abstract. The invention of self-shielding copper substrate neutron guides is described, along with the rationale behind the development, and the realisation of commercial supply. The relative advantages with respect to existing technologies are quantified. These include ease of manufacture, long lifetime, increased thermal conductivity, and enhanced fast neutron attenuation in the keV-MeV energy range. Whilst the activation of copper is initially higher than for other material options, for the full energy spectrum, many of the isotopes are short-lived, so that for realistic maintenance access times the radiation dose to workers is expected to be lower than steel and in the lowest zoning category for radiation safety outside the spallation target monolith. There is no impact on neutron reflectivity performance relative to established alternatives, and the manufacturing cost is similar to other polished metal arXiv:2104.02453v2 [physics.ins-det] 16 Apr 2021 substrates. -
Polarizing Multilayer Neutron Mirrors
Die approbierte Originalversion dieser Diplom-/ Masterarbeit ist in der Hauptbibliothek der Tech- nischen Universität Wien aufgestellt und zugänglich. http://www.ub.tuwien.ac.at The approved original version of this diploma or master thesis is available at the main library of the Vienna University of Technology. http://www.ub.tuwien.ac.at/eng DIPLOMARBEIT Polarizing Multilayer Neutron Mirrors zur Erlangung des akademischen Grades Diplom-Ingenieur im Rahmen des Studiums Technische Physik eingereicht von Martin Wess Matrikelnummer 01325756 ausgef¨uhrtam Atominstitut der Fakult¨atf¨urPhysik der Technischen Universit¨atWien in Zusammenarbeit mit der Universit¨atLink¨oping Betreuung Betreuer: Univ.Prof. Dipl.-Ing. Dr.techn. Gerald Badurek Mitwirkung: Ass.Prof. Dipl.-Ing. Dr.techn. Erwin Jericha Mitwirkung: Assoc.Prof. Dr.techn. Fredrik Eriksson (Link¨oping) Wien, 01.04.2019 (Unterschrift Verfasser) (Unterschrift Betreuer) Zusammenfassung Diese Diplomarbeit beschreibt Prinzip, Design, Herstellung und Charakterisierung von polarisierenden Vielschicht-Neutronenspiegeln. Das Hauptaugenmerk wurde auf die Optimierung der Umgebungsparameter w¨ahrendder Herstellung gelegt, um m¨oglichst ideale Grenzfl¨achen zu generieren. Die Grenzfl¨achen sollen minimale Rauheit und Mischung zwischen den Schichten aufweisen, um Neutronenspiegel mit hoher Qualit¨atzu erhalten. Um polarisierende Vielschicht-Neutronenspiegel herzustellen, muss eines der beiden gew¨ahlten Materialien ferromagnetisch sein. Außerdem ist es wichtig, dass das nukleare Fermi-Pseudopotential f¨urden niedrigeren Spinzustand des ferromag- netischen Partners m¨oglichst gleich groß ist wie das des unmagnetischen Materi- als. Cobalt (Co) und Titan (Ti) erf¨ullendiese Voraussetzungen und wurden daher als Materialien gew¨ahlt.Der Einfluss der Beimischung von isotopangereichertem, 11 schwach Neutron-absorbierendem Borcarbid ( B4C) auf die Qualit¨atder Grenz- fl¨achen wurde gr¨undlich untersucht. -
Neutron-Antineutron Oscillation Experiments
Neutron-AntineutronNeutron-Antineutron OscillationOscillation Experiments:Experiments: WhatWhat HaveHave WeWe LearnedLearned atat thethe Workshop?Workshop? W. M. Snow Indiana University/CEEM Project X Workshop Why ΔB=2? (theory/phenomenology) Neutron-antineutron oscillations in nuclei:theory and experiment Free neutron oscillations: experimental requirements @Project X Thanks to co-conveners: Chris Quigg (FNAL), Albert Young (NC State) Neutron-AntineutronNeutron-Antineutron Oscillations:Oscillations: SpeakerSpeaker ListList (from(from Germany,Germany, Georgia,Georgia, India,India, Japan,Japan, US)US) Speaker Subject R. Mohapathra, Maryland theory/phenomonology M. Snow, Indiana various G. Greene, ORNL/Tennessee R&D needs I. Gogoladze, Bartol/Delaware theory/phenomonology M. Chen, Irvine leptogenesis K. Babu, Oklahoma State theory/phenomonology M. Stavenga, FNAL theory M. Buchoff, LLNL theory/lattice E. Kearns, Boston experiment/nnbar in nuclei A. Vainshtein, Minnesota theory/nnbar in nuclei Y. Kamyshkov, Tennessee experiment options R. Tayloe, Indiana detectors K. Ganezer, CSUDH nnbar in nuclei D. Dubbers, Heidelberg ILL experiment T. Gabriel, ORNL/Tennessee SNS 1MW target G. Muhrer, LANL 1MW target/moderator design H. Shimizu, Nagoya neutron supermirror optics C-Y Liu, Indiana (also for D. Baxter, Indiana) moderator experiments/simulations S. Banerjee, Tata Institute detectors Neutron-Antineutron Oscillations: Formalism " n% ! = n-nbar state vector α≠0 allows oscillations #$ n&' " En ( % H = $ ' Hamiltonian of n-nbar system # ( E n & p2 p2 En = mn + + Un ; En = mn + + Un 2mn 2mn Note : • ( real (assuming T) • mn = mn (assuming CPT) U U in matter and in external B [ n n from CPT] • n ) n µ( ) = *µ( ) Neutron-Antineutron transition probability # E + V ! & ! 2 + ! 2 + V 2 . For H = P (t) = * sin2 - t 0 % E V ( n)n 2 2 $ ! " ' ! + V ,- ! /0 where V is the potential difference for neutron and anti-neutron.