Paranemic Crossover DNA: There and Back Again Xing Wang,*,† Arun Richard Chandrasekaran,*,‡ Zhiyong Shen,§ Yoel P
Total Page:16
File Type:pdf, Size:1020Kb
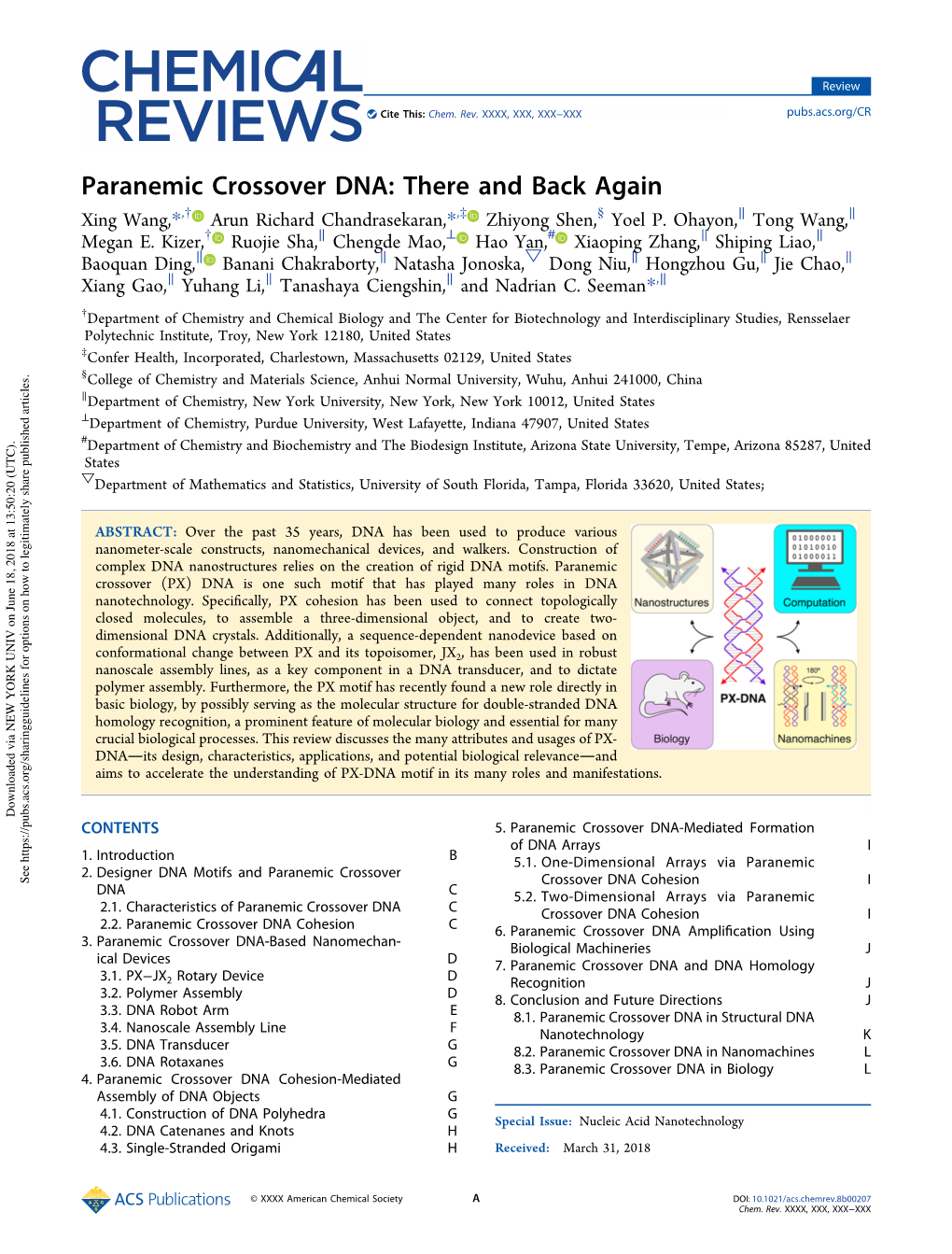
Load more
Recommended publications
-
Programming Structured DNA Assemblies to Probe Biophysical Processes
Programming Structured DNA Assemblies to Probe Biophysical Processes The MIT Faculty has made this article openly available. Please share how this access benefits you. Your story matters. Citation Wamhoff, Eike-Christian et al. “Programming Structured DNA Assemblies to Probe Biophysical Processes.” Annual Review of Biophysics 48 (2019): 395-419 © 2019 The Author(s) As Published 10.1146/ANNUREV-BIOPHYS-052118-115259 Publisher Annual Reviews Version Author's final manuscript Citable link https://hdl.handle.net/1721.1/125280 Terms of Use Creative Commons Attribution-Noncommercial-Share Alike Detailed Terms http://creativecommons.org/licenses/by-nc-sa/4.0/ HHS Public Access Author manuscript Author ManuscriptAuthor Manuscript Author Annu Rev Manuscript Author Biophys. Author Manuscript Author manuscript; available in PMC 2020 February 22. Published in final edited form as: Annu Rev Biophys. 2019 May 06; 48: 395–419. doi:10.1146/annurev-biophys-052118-115259. Programming Structured DNA Assemblies to Probe Biophysical Processes Eike-Christian Wamhoff, James L. Banal, William P. Bricker, Tyson R. Shepherd, Molly F. Parsons, Rémi Veneziano, Matthew B. Stone, Hyungmin Jun, Xiao Wang, Mark Bathe Department of Biological Engineering, Massachusetts Institute of Technology, Cambridge, Massachusetts 02139, USA; Abstract Structural DNA nanotechnology is beginning to emerge as a widely accessible research tool to mechanistically study diverse biophysical processes. Enabled by scaffolded DNA origami in which a long single strand of DNA is weaved throughout an entire target nucleic acid assembly to ensure its proper folding, assemblies of nearly any geometric shape can now be programmed in a fully automatic manner to interface with biology on the 1–100-nm scale. -
Artificial RNA Motifs Expand the Programmable Assembly Between
biology Article Artificial RNA Motifs Expand the Programmable Assembly between RNA Modules of a Bimolecular Ribozyme Leading to Application to RNA Nanostructure Design Md. Motiar Rahman 1,2 ID , Shigeyoshi Matsumura 1 and Yoshiya Ikawa 1,2,* ID 1 Department of Chemistry, Graduate School of Science and Engineering, University of Toyama, Gofuku 3190, Toyama 9308555, Japan; [email protected] (Md.M.R.); [email protected] (S.M.) 2 Graduate School of Innovative Life Science, University of Toyama, Gofuku 3190, Toyama 9308555, Japan * Correspondence: [email protected]; Tel.: +81-76-445-6599 Academic Editor: Jukka Finne Received: 17 September 2017; Accepted: 25 October 2017; Published: 30 October 2017 Abstract: A bimolecular ribozyme consisting of a core ribozyme (DP5 RNA) and an activator module (P5abc RNA) has been used as a platform to design assembled RNA nanostructures. The tight and specific assembly between the P5abc and DP5 modules depends on two sets of intermodule interactions. The interface between P5abc and DP5 must be controlled when designing RNA nanostructures. To expand the repertoire of molecular recognition in the P5abc/DP5 interface, we modified the interface by replacing the parent tertiary interactions in the interface with artificial interactions. The engineered P5abc/DP5 interfaces were characterized biochemically to identify those suitable for nanostructure design. The new interfaces were used to construct 2D-square and 1D-array RNA nanostructures. Keywords: catalytic RNA; group I intron; ribozyme; RNA nanostructure; RNA nanotechnology 1. Introduction Nanometer-sized biomolecules and their assemblies with precisely controlled shapes are attractive materials for the expanding field of nanobioscience, which includes nanomedicine and nanobiotechnology. -
Abstract Booklet
Abstract Booklet ONLINE EVENT 26th International Conference on DNA Computing and Molecular Programming 14–17 September 2020 IOP webinars for the physics community dna26.iopconfs.org/ Contents Committees 2 Sponsors 3 Proceedings 3 Programme 4 Poster programme 8 Keynote presentations 11 Contributed presentations 17 Poster sessions 48 1 Committees Chairs • Andrew Phillips, Microsoft Research, Cambridge, UK • Andrew Turberfield, Department of Physics, University of Oxford, UK Programme Chairs • Cody Geary, Interdisciplinary Nanoscience Centre, University of Aarhus, Denmark • Matthew Patitz, Department of Computer Science and Computer Engineering, University of Arkansas Steering Committee • Luca Cardelli, Computer Science, Oxford University, UK • Anne Condon (Chair), Computer Science, University of British Columbia, Canada • Masami Hagiya, Computer Science, University of Tokyo, Japan • Natasha Jonoska, Mathematics, University of Southern Florida, USA • Lila Kari, Computer Science, University of Waterloo, Canada • Chengde Mao, Chemistry, Purdue University, USA • Satoshi Murata, Robotics, Tohoku University, Japan • John H. Reif, Computer Science, Duke University, USA • Grzegorz Rozenberg, Computer Science, University of Leiden, The Netherlands • Rebecca Schulman, Chemical and Biomolecular Engineering, Johns Hopkins University, USA • Nadrian C. Seeman, Chemistry, New York University, USA • Friedrich Simmel, Physics, Technical University Munich, Germany • David Soloveichik, Electrical and Computer Engineering, The University of Texas at Austin, -
RNA-DNA Hybrid Origami: Folding a Long RNA Single Strands Into Complex Nanostructures Pengfei Wang,A Seung Hyeon Ko,B± Cheng Tian,B Chenhui Hao B & Chengde Mao*B
Electronic Supplementary Material (ESI) for Chemical Communications This journal is © The Royal Society of Chemistry 2013 # Supplementary Material (ESI) for Chemical Communications # This journal is (c) The Royal Society of Chemistry 2010 RNA-DNA Hybrid Origami: Folding a Long RNA Single Strands into Complex Nanostructures Pengfei Wang,a Seung Hyeon Ko,b± Cheng Tian,b Chenhui Hao b & Chengde Mao*b Supplementary Information Materials and Methods Oligonucleotides: Forward primer (P1): 5'-ttctaatacgactcactataggtctgacagttaccaat-3' (the T7 promoter sequence is underlined.); Reverse primer (P2): 5'-gacgaaagggcctcgt-3'. The sequences for RNA scaffold and DNA staples are given in Figure S1. Both primers and DNA staple strands were purchased from Integrated DNA technology (IDT) and used without further purification. PCR amplification of DNA template: The dsDNA template containing T7 promoter sequence was prepared by polymerase chain reaction (PCR) with a DNA plasmid pUC19 (New England Biolabs), primers (P1 and P2), and GoTaq Flexi DNA polymerase (Promega). In vitro RNA transcription: AmpliScribe™ T7-Flash™ Transcription Kit (Epicentre) was used to transcribe single-stranded RNA from DNA template, following the protocol provided with the kit. Briefly, a total of 20 uL mixture, including DNA template, ribonucleotides, RNase inhibitor together with T7-Flash RNA polymerase, was prepared in the provided reaction buffer, and then incubated at 42 °C for 120 mins. Then, transcription product was treated by DNase I to remove DNA template by incubating at 37 °C for 15 mins. Quantification of RNA scaffold: Purified RNA strands were quantified by a UV-Vis spectrophotometer. RNA strands without purification after in vitro transcription were indirectly quantified through agarose gel electrophoresis by using purified RNA as the calibrator. -
Enhanced Anticoagulation Activity of Functional RNA Origami
bioRxiv preprint doi: https://doi.org/10.1101/2020.09.29.319590; this version posted October 1, 2020. The copyright holder for this preprint (which was not certified by peer review) is the author/funder. All rights reserved. No reuse allowed without permission. Enhanced Anticoagulation Activity of Functional RNA Origami Abhichart Krissanaprasit, Carson M. Key, Kristen Froehlich, Sahil Pontula, Emily Mihalko, Daniel M. Dupont, Ebbe S. Andersen, Jørgen Kjems, Ashley C. Brown, and Thomas H. LaBean* Dr. A Krissanaprasit, C. M. Key, Prof. T. H. LaBean Department of Materials Science and Engineering, College of Engineering, North Carolina State University, Raleigh, NC, 27695, USA. E-mail: [email protected] K. Froehlich, E. Mihalko, Prof. A. C. Brown Joint Department of Biomedical Engineering, College of Engineering, North Carolina State University and University of North Carolina - Chapel Hill, Raleigh, NC, 27695, USA. S. Pontula William G. Enloe High School, Raleigh, NC 27610, USA Dr. D. M. Dupont, Prof. E. S. Andersen, Prof. J. Kjems Interdisciplinary Nanoscience Center (iNANO), Aarhus University, 8000 Aarhus C, Denmark Prof. A. C. Brown, Prof. T. H. LaBean Comparative Medicine Institute, North Carolina State University and University of North Carolina - Chapel Hill, Raleigh, NC, 27695, USA. Keywords: anticoagulant, RNA origami, nucleic acid, RNA nanotechnology, aptamer, direct thrombin inhibitor, reversal agent 1 bioRxiv preprint doi: https://doi.org/10.1101/2020.09.29.319590; this version posted October 1, 2020. The copyright holder for this preprint (which was not certified by peer review) is the author/funder. All rights reserved. No reuse allowed without permission. Abstract Anticoagulants are commonly utilized during surgeries and to treat thrombotic diseases like stroke and deep vein thrombosis. -
Co-Transcriptional Folding of a Bio-Orthogonal Fluorescent Scaffolded RNA Origami
bioRxiv preprint doi: https://doi.org/10.1101/864678; this version posted December 5, 2019. The copyright holder for this preprint (which was not certified by peer review) is the author/funder. All rights reserved. No reuse allowed without permission. 1 bioRxiv preprint doi: https://doi.org/10.1101/864678; this version posted December 5, 2019. The copyright holder for this preprint (which was not certified by peer review) is the author/funder. All rights reserved. No reuse allowed without permission. Co-transcriptional folding of a bio-orthogonal fluorescent scaffolded RNA origami Emanuela Torelli1,*, Jerzy W. Kozyra1,§, Ben Shirt-Ediss1, Luca Piantanida2,^, Kislon Voïtchovsky2, and Natalio Krasnogor1,* 1Interdisciplinary Computing and Complex BioSystems (ICOS), Centre for Synthetic Biology and Bioeconomy (CSBB), Devonshire Building, Newcastle University, Newcastle upon Tyne, NE1 7RX, United Kingdom 2Department of Physics, Durham University, Durham, DH1 3LE, United Kingdom §Present Address: Nanovery Ltd, 85 Great Portland Street, First Floor, London, W1W 7LT, United Kingdom ^Present Address: Micron School of Materials Science & Engineering, Boise State University, Boise, ID 83725, USA *[email protected], [email protected] 2 bioRxiv preprint doi: https://doi.org/10.1101/864678; this version posted December 5, 2019. The copyright holder for this preprint (which was not certified by peer review) is the author/funder. All rights reserved. No reuse allowed without permission. ABSTRACT The scaffolded origami technique has provided an attractive tool for engineering nucleic acid nanostructures. This paper demonstrates scaffolded RNA origami folding in vitro in which all components are transcribed simultaneously in a single-pot reaction. Double-stranded DNA sequences are transcribed by T7 RNA polymerase into scaffold and staple strands able to correctly fold in high yield into the nanoribbon. -
Title Preparation of Chemically Modified RNA Origami
CORE Metadata, citation and similar papers at core.ac.uk Provided by Kyoto University Research Information Repository Preparation of chemically modified RNA origami Title nanostructures. Endo, Masayuki; Takeuchi, Yosuke; Emura, Tomoko; Hidaka, Author(s) Kumi; Sugiyama, Hiroshi Citation Chemistry - A European Journal (2014), 20(47): 15330-15333 Issue Date 2014-10-14 URL http://hdl.handle.net/2433/198828 This is the peer reviewed version of the following article: Endo, M., Takeuchi, Y., Emura, T., Hidaka, K. and Sugiyama, H. (2014), Preparation of Chemically Modified RNA Origami Nanostructures. Chem. Eur. J., 20: 15330‒15333, which has Right been published in final form at http://dx.doi.org/10.1002/chem.201404084. This article may be used for non-commercial purposes in accordance with Wiley Terms and Conditions for Self-Archiving.; 許諾条件により本 文ファイルは2015-10-14に公開. Type Journal Article Textversion author Kyoto University COMMUNICATION Preparation of Chemically Modified RNA Origami Nanostructures Masayuki Endo,*[a][c] Yosuke Takeuchi,[b] Tomoko Emura,[b] Kumi Hidaka,[a] and Hiroshi Sugiyama*[a][b][c] Abstract: RNA molecules potentially have structural diversity and scaffold and staple RNA strands (Figure 1a). The RNA origami functionalities, and it should be required to organize the structures for a 7-helix bundled planar structure (7HB-tile) was designed and functions in a designed fashion. We developed the method to with a geometry of 33 bp per three turns in the adjacent design and prepare RNA nanostructures by employing DNA origami crossover points, which corresponds to a helical pitch with 11 strategy. RNA transcripts were used as a single-stranded RNA bp/turn. -
Control of RNA Function by Conformational Design
DISSERTATION / DOCTORAL THESIS Titel der Dissertation /Title of the Doctoral Thesis Control of RNA function by conformational design verfasst von / submitted by Mag. rer. nat. Stefan Badelt angestrebter akademischer Grad / in partial fulfilment of the requirements for the degree of Doctor of Philosophy (PhD) Wien, 2016 / Vienna, 2016 Studienkennzahl lt. Studienblatt / A 794 685 490 degree programme code as it appears on the student record sheet: Dissertationsgebiet lt. Studienblatt / Molekulare Biologie field of study as it appears on the student record sheet: Betreut von / Supervisor: Univ.-Prof. Dipl.-Phys. Dr. Ivo Hofacker Design landscapes >Output Input AGGAACAGUCGCACU ACCCACCUCGACAUC AGCAACA GUAAAUCAAAUUGGA AGGAUCU ACUGAAGCCCUUGGU AGGAUCA CUGGAGUCACCAGGG objective function AGGAACA GGUUUACGUACUACU design Energy landscapes 0.7 0.7 >Input -8.0 Output 3.9 3.7 1.1 2.8 1.2 1.3 2.3 0.8 AGGAACAGUCGCACU 3.5 2.6 2.6 2.8 3.9 2.3 -10.0 3.0 4.2 1.2 0.7 2.3 1.2 4.4 1.3 0.599999 1.3 2.4 1.0 0.8 100 1.5 2.0 1.2 0.8 97 98 99 2.8 1.4 1.0 94 95 93 96 92 2.3 0.7 2.6 0.8 91 1.5 1.3 2.0 2.6 1.6 89 90 1.9 0.8 2.0 86 88 87 2.9 2.3 1.9 1.3 1.3 ACCCACCUCGACAUC 0.6 83 85 84 82 2.6 1.0 0.8 0.8 77 81 79 78 80 0.7 76 1.9 74 75 73 1.5 72 0.7 -12.0 0.8 0.8 70 71 1.1 0.799999 1.2 0.8 69 68 0.8 0.8 0.8 63 65 64 66 67 0.9 1.2 1.2 59 60 58 61 62 1.2 1.4 1.7 1.2 55 54 57 56 3.1 3.0 4.2 52 53 51 49 50 0.7 1.0 45 44 46 47 48 42 43 41 3.5 1.0 38 39 40 1.3 0.6 2.6 1.3 0.8 0.8 0.8 GUAAAUCAAAUUGGA 37 35 36 34 32 33 31 30 28 29 27 1.2 0.8 24 25 26 23 -14.0 22 20 21 2.4 17 -
Branched Kissing Loops for the Construction of Diverse RNA Homooligomeric 2 Nanostructures 3 Di Liu1, Cody W
1 Branched kissing loops for the construction of diverse RNA homooligomeric 2 nanostructures 3 Di Liu1, Cody W. Geary2,3, Gang Chen1, Yaming Shao4, Mo Li5, Chengde Mao5, Ebbe S. Andersen2, Joseph A. Piccirilli1,4, 4 Paul W. K. Rothemund3, Yossi Weizmann1,* 5 1Department of Chemistry, the University of Chicago, Chicago, Illinois 60637, USA 6 2Interdisciplinary Nanoscience Center and Department of Molecular Biology and Genetics, Aarhus University, 8000 Aarhus, Denmark 7 3Bioengineering, Computational + Mathematical Sciences, and Computation & Neural Systems, California Institute of Technology, 8 Pasadena, CA 91125, USA 9 4Department of Biochemistry and Molecular Biology, the University of Chicago, Chicago, Illinois 60637, USA 10 5Department of Chemistry, Purdue University, West Lafayette, IN 47907, USA 11 *Correspondence to: [email protected] (Y. W.) 12 13 Summary figure 14 15 16 17 This study presents a robust homooligomeric self-assembly system based on RNA and DNA tiles that are 18 folded from a single-strand of nucleic acid and assemble via a novel artificially designed branched 19 kissing-loops (bKL) motif. By adjusting the geometry of individual tiles, we have constructed a total of 16 20 different structures that demonstrate our control over the curvature, torsion, and number of helices of 21 assembled structures. Furthermore, bKL-based tiles can be assembled cotranscriptionally, and can be 22 expressed in living bacterial cells. 23 24 Branched kissing loops for the construction of diverse RNA homooligomeric 25 nanostructures -
Synthesis of DNA Origami Scaffolds: Current and Emerging Strategies
molecules Review Synthesis of DNA Origami Scaffolds: Current and Emerging Strategies 1, 1, 1, 1 1 Joshua Bush y , Shrishti Singh y , Merlyn Vargas y , Esra Oktay , Chih-Hsiang Hu and Remi Veneziano 1,2,* 1 Volgenau School of Engineering, Department of Bioengineering, George Mason University, Fairfax, VA 22030, USA; [email protected] (J.B.); [email protected] (S.S.); [email protected] (M.V.); [email protected] (E.O.); [email protected] (C.-H.H.) 2 Institute for Advanced Biomedical Research, George Mason University, Manassas, VA 20110, USA * Correspondence: [email protected] These authors contributed equally to this work. y Academic Editor: Kirill A. Afonin Received: 1 July 2020; Accepted: 24 July 2020; Published: 26 July 2020 Abstract: DNA origami nanocarriers have emerged as a promising tool for many biomedical applications, such as biosensing, targeted drug delivery, and cancer immunotherapy. These highly programmable nanoarchitectures are assembled into any shape or size with nanoscale precision by folding a single-stranded DNA scaffold with short complementary oligonucleotides. The standard scaffold strand used to fold DNA origami nanocarriers is usually the M13mp18 bacteriophage’s circular single-stranded DNA genome with limited design flexibility in terms of the sequence and size of the final objects. However, with the recent progress in automated DNA origami design—allowing for increasing structural complexity—and the growing number of applications, the need for scalable methods to produce custom scaffolds has become crucial to overcome the limitations of traditional methods for scaffold production. Improved scaffold synthesis strategies will help to broaden the use of DNA origami for more biomedical applications. -
Algorithmic Design of Cotranscriptionally Folding 2D RNA Origami Structures?
Algorithmic Design of Cotranscriptionally Folding 2D RNA Origami Structures? Abdulmelik Mohammed1, Pekka Orponen1, and Sachith Pai1 Aalto University, Department of Computer Science FI-00076 Aalto, Finland [email protected], [email protected], [email protected] Abstract. We address a biochemical folding obstacle of \polymerase trapping" that arises in the remarkable RNA origami tile design frame- work of Geary, Rothemund and Andersen (Science 2014). We present a combinatorial formulation of this obstacle, together with an optimisa- tion procedure that yields designs minimising the risk of encountering the corresponding topological trap in the tile folding phase. The procedure has been embedded in an automated software pipeline, and we provide examples of designs produced by the software, including an optimised version of the RNA smiley-face tile proposed by Geary and Andersen (DNA 2014). Keywords: RNA origami, RNA tiles, RNA nanotechnology, Rational design, Cotranscriptional folding, Grid graphs, Spanning trees 1 Introduction Following the introduction of Paul Rothemund's DNA origami technique in 2006 [14], the research area of DNA nanotechnology [15] has made rapid progress in the rational design of highly complex 2D and 3D DNA nanostructures and their applications [10, 12, 13, 16, 18]. In the past few years, there has also been increasing interest in using RNA, rather than DNA, as the fundamental construc- tion material for similar purposes [5, 7{9]. One great appeal of this alternative is that while the production of designed DNA nanostructures typically proceeds by a multi-stage laboratory protocol that involves synthesising the requisite nucleic acid strands and hybridising them together in a thermally controlled process, RNA nanostructures can in principle be produced in quantity by the natu- ral process of polymerase transcription from a representative DNA template, isothermally at room temperature, in vitro and eventually in vivo. -
Delivering DNA Origami to Cells
Review For reprint orders, please contact: [email protected] Delivering DNA origami to cells Dhanasekaran Balakrishnan‡,1,2, Gerrit D Wilkens‡,1,2 & Jonathan G Heddle*,1 1Bionanoscience & Biochemistry Laboratory, Malopolska Centre of Biotechnology, Jagiellonian University, Gronostajowa 7A, 30–387 Krakow, Poland 2Postgraduate School of Molecular Medicine; Zwirki˙ i Wigury 61, 02–091 Warsaw, Poland *Author for correspondence: [email protected] ‡Authors contributed equally DNA nanotechnology research has long-held promise as a means of developing functional molecules ca- pable of delivery to cells. Recent advances in DNA origami have begun to realize this potential but is still at the earliest stage and a number of hurdles remain. This review focuses on progress in addressing these hurdles and considers some of the challenges still outstanding. These include stability of such structures necessary to reach target cells after administration; methods of cell targeting and uptake; strategies to avoid or escape endosomes and techniques for achieving specific subcellular localization. Finally, the func- tionality that can be expected once DNA origami structures reach their final intracellular targets will be considered. Lay abstract: The DNA origami technique allows ‘nanorobots’ to be made from DNA. These have the potential to work as ‘smart’ drugs but only if they can be successfully delivered to cells. Here, we review progress in achieving this goal. First draft submitted: 15 November 2018; Accepted for publication: 23 January 2019; Published online: 22 March 2019 Keywords: cell delivery • cell targeting • DNA origami • DNA nanotechnology • nanorobots • smart medicines DNA nanotechnology can trace its roots back to Nadrian Seeman, who proposed that by introducing unique sequences in the arms of Holliday junctions, immobile branched DNA junctions could be constructed, which could be used as building blocks to assemble higher order structures such as polyhedra [1].