A Study on an Altitudinal Gradient Investigating the Potential Effects of Climate Change on Fynbos and the Fynbos-Succulent Karoo Boundary
Total Page:16
File Type:pdf, Size:1020Kb
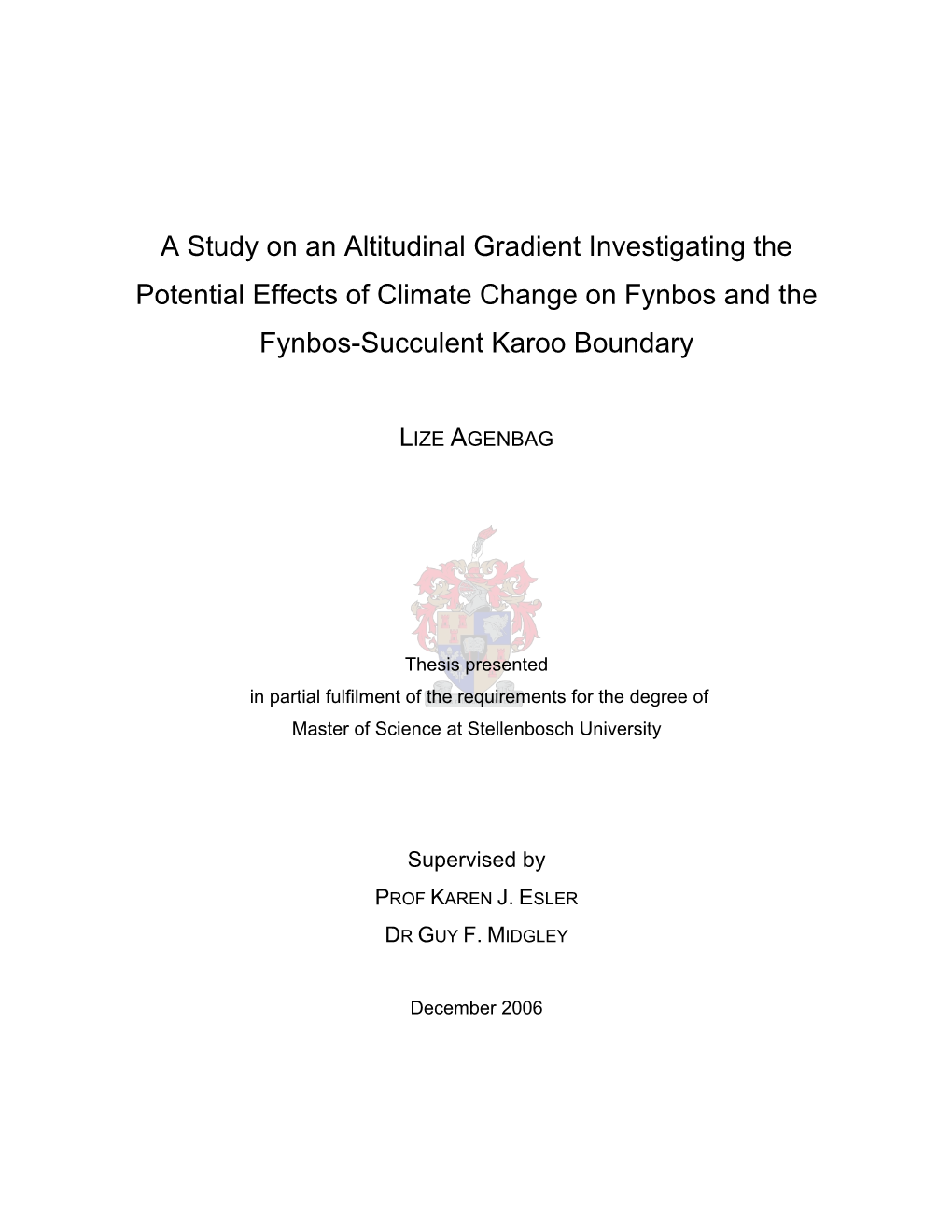
Load more
Recommended publications
-
Progress on Boneseed (Chrysanthemoides Monilifera Ered for Biological Control of Boneseed Subsp
Plant Protection Quarterly Vol.23(1) 2008 29 Chrysanthemoides seed fl ies Three Mesoclanis spp. have been consid- Progress on boneseed (Chrysanthemoides monilifera ered for biological control of boneseed subsp. monilifera (L.) Norlindh) biological control: and bitou bush in Australia (Edwards and Brown 1997; Neser and Morris 1985). These the boneseed leaf buckle mite Aceria (Keifer) sp., the fl ies lay their eggs into Chrysanthemoides fl owerheads and the larvae can destroy lacy-winged seed fl y Mesoclanis magnipalpis Bezzi substantial proportions of developing and the boneseed rust Endophyllum osteospermi ovules, thus suppressing seed production. When introduced to Australia in 1996, the (Doidge) A.R.Wood bitou seed fl y (Mesoclanis polana Munro) (BSF) rapidly colonized almost the entire A B T.B. Morley and L. Morin range of bitou bush (Edwards et al. 1999). A Department of Primary Industries, PO Box 48, Frankston, Victoria 3199, However, in South Africa the BSF does not Australia. utilize boneseed and prevails at latitudes B CSIRO Entomology, GPO Box 1700, Canberra, ACT 2601, Australia. much closer to the equator than the more southerly bitou bush infestations in Aus- tralia. The BSF was therefore considered unlikely to effectively suppress seed pro- duction of Australian boneseed or the more Introduction stimulates dispersal. Dispersive mites can southerly bitou bush infestations, and this So far six exotic organisms have been re- walk to adjacent uncolonized shoot tips or appears to be true (Robin Adair personal leased in Australia as potential biological can be wind-dispersed to other boneseed communication and Morley unpublished control agents for the environmental weed plants. -
Wide Variation in Post-Emergence Desiccation Tolerance of Seedlings of Fynbos Proteoid Shrubs ⁎ P.J
Available online at www.sciencedirect.com South African Journal of Botany 80 (2012) 110–117 www.elsevier.com/locate/sajb Wide variation in post-emergence desiccation tolerance of seedlings of fynbos proteoid shrubs ⁎ P.J. Mustart a, A.G. Rebelo b, J. Juritz c, R.M. Cowling a, a Botany Department, Nelson Mandela Metropolitan University, P.O. Box 77000, Port Elizabeth 6301, South Africa b Kirstenbosch Research Centre, South African National Biodiversity Institute, Private Bag X7, Claremont 7735, South Africa c Department of Statistical Science, University of Cape Town, Private Bag, Rondebosch 7700, South Africa Received 20 February 2012; received in revised form 13 March 2012; accepted 21 March 2012 Abstract Fynbos Proteaceae that are killed by fire and bear their seeds in serotinous cones (proteoids), are entirely dependent on seedling recruitment for persistence. Hence, the regeneration phase represents a vulnerable stage of the plant life cycle. In laboratory-based experiments we investigated the effect of desiccation on the survival of newly emerged seedlings of 23 proteoid species (Leucadendron and Protea) occurring in a wide variety of fynbos habitats. We tested the hypothesis that species of drier habitats would be more tolerant of desiccation than those from more moist areas. Results showed that with no desiccation treatment, or with desiccation prior to radicle emergence, all species germinated to high levels. However, with desiccation treatments imposed after radicle emergence, there were significant declines in seedling emergence after subsequent re-wetting. Furthermore, other than three species that grow in waterlogged habitats, germination responses could not be reliably modeled as a function of soil moisture variables. -
Chrysanthemoides Monilifera Ssp
MANAGEMENT OF BONESEED (CHRYSANTHEMOIDES MONILIFERA SSP. MONILIFERA) (L.) T. NORL. USING FIRE, HERBICIDES AND OTHER TECHNIQUES IN AUSTRALIAN WOODLANDS Rachel L. Melland Thesis submitted for the degree of Doctor of Philosophy School of Agriculture, Food and Wine University of Adelaide August 2007 Table of Contents TABLE OF CONTENTS ....................................................................................................... II ABSTRACT ............................................................................................................................ VI DECLARATION ................................................................................................................ VIII ACKNOWLEDGEMENTS .................................................................................................. IX CHAPTER 1: INTRODUCTION ............................................................................................ 1 1.1 AIMS OF THIS THESIS .......................................................................................................... 3 CHAPTER 2: LITERATURE REVIEW ............................................................................... 5 2.1 PROCESSES OF NATIVE ECOSYSTEM DEGRADATION ............................................................ 5 2.2 GLOBAL PLANT INVASIONS – ECOSYSTEM DEGRADING PROCESSES .................................... 6 2.3 THE ENVIRONMENTAL WEED PROBLEM IN AUSTRALIA ..................................................... 10 2.4 CAUSES AND PROCESSES OF INVASIVENESS ..................................................................... -
Pathogens Associated with Diseases. of Protea, Leucospermum and Leucadendron Spp
PATHOGENS ASSOCIATED WITH DISEASES. OF PROTEA, LEUCOSPERMUM AND LEUCADENDRON SPP. Lizeth Swart Thesis presented in partial fulfillment of the requirements for the degree of Master of Science in Agriculture at the University of Stellenbosch Supervisor: Prof. P. W. Crous Decem ber 1999 Stellenbosch University https://scholar.sun.ac.za DECLARATION 1, the undersigned, hereby declare that the work contained in this thesis is my own original work and has not previously in its entirety or in part been submitted at any university for a degree. SIGNATURE: DATE: Stellenbosch University https://scholar.sun.ac.za PATHOGENS ASSOCIATED WITH DISEASES OF PROTEA, LEUCOSPERMUM ANDLEUCADENDRONSPP. SUMMARY The manuscript consists of six chapters that represent research on different diseases and records of new diseases of the Proteaceae world-wide. The fungal descriptions presented in this thesis are not effectively published, and will thus be formally published elsewhere in scientific journals. Chapter one is a review that gives a detailed description of the major fungal pathogens of the genera Protea, Leucospermum and Leucadendron, as reported up to 1996. The pathogens are grouped according to the diseases they cause on roots, leaves, stems and flowers, as well as the canker causing fungi. In chapter two, several new fungi occurring on leaves of Pro tea, Leucospermum, Telopea and Brabejum collected from South Africa, Australia or New Zealand are described. The following fungi are described: Cladophialophora proteae, Coniolhyrium nitidae, Coniothyrium proteae, Coniolhyrium leucospermi,Harknessia leucospermi, Septoria prolearum and Mycosphaerella telopeae spp. nov. Furthermore, two Phylloslicla spp., telopeae and owaniana are also redecribed. The taxonomy of the Eisinoe spp. -
Biodiversity and Ecology of Critically Endangered, Rûens Silcrete Renosterveld in the Buffeljagsrivier Area, Swellendam
Biodiversity and Ecology of Critically Endangered, Rûens Silcrete Renosterveld in the Buffeljagsrivier area, Swellendam by Johannes Philippus Groenewald Thesis presented in fulfilment of the requirements for the degree of Masters in Science in Conservation Ecology in the Faculty of AgriSciences at Stellenbosch University Supervisor: Prof. Michael J. Samways Co-supervisor: Dr. Ruan Veldtman December 2014 Stellenbosch University http://scholar.sun.ac.za Declaration I hereby declare that the work contained in this thesis, for the degree of Master of Science in Conservation Ecology, is my own work that have not been previously published in full or in part at any other University. All work that are not my own, are acknowledge in the thesis. ___________________ Date: ____________ Groenewald J.P. Copyright © 2014 Stellenbosch University All rights reserved ii Stellenbosch University http://scholar.sun.ac.za Acknowledgements Firstly I want to thank my supervisor Prof. M. J. Samways for his guidance and patience through the years and my co-supervisor Dr. R. Veldtman for his help the past few years. This project would not have been possible without the help of Prof. H. Geertsema, who helped me with the identification of the Lepidoptera and other insect caught in the study area. Also want to thank Dr. K. Oberlander for the help with the identification of the Oxalis species found in the study area and Flora Cameron from CREW with the identification of some of the special plants growing in the area. I further express my gratitude to Dr. Odette Curtis from the Overberg Renosterveld Project, who helped with the identification of the rare species found in the study area as well as information about grazing and burning of Renosterveld. -
Image Identification of Protea Species with Attributes and Subgenus Scaling
Image identification of Protea species with attributes and subgenus scaling Peter Thompson Willie Brink Stellenbosch University Stellenbosch University [email protected] [email protected] Abstract The flowering plant genus Protea is a dominant repre- sentative for the biodiversity of the Cape Floristic Region in South Africa, and from a conservation point of view im- portant to monitor. The recent surge in popularity of crowd- sourced wildlife monitoring platforms presents both chal- lenges and opportunities for automatic image based species identification. We consider the problem of identifying the Protea species in a given image with additional (but op- tional) attributes linked to the observation, such as loca- tion and date. We collect training and test data from a crowd-sourced platform, and find that the Protea identifi- Figure 1. Different species of Protea, such as Protea neriifolia cation problem is exacerbated by considerable inter-class and Protea laurifolia shown here, can exhibit considerable visual similarity, data scarcity, class imbalance, as well as large similarity. variations in image quality, composition and background. Our proposed solution consists of three parts. The first part incorporates a variant of multi-region attention into a pre- important for understanding species populations [3] in the trained convolutional neural network, to focus on the flow- midst of issues like global warming, pollution and poach- erhead in the image. The second part performs coarser- ing. The crowd-sourced platform iNaturalist for example grained classification on subgenera (superclasses) and then allows users to upload observations of wildlife, which typ- rescales the output of the first part. The third part con- ically include images, locations, dates, and identifications ditions a probabilistic model on the additional attributes that can be verified by fellow users [31]. -
Sand Mine Near Robertson, Western Cape Province
SAND MINE NEAR ROBERTSON, WESTERN CAPE PROVINCE BOTANICAL STUDY AND ASSESSMENT Version: 1.0 Date: 06 April 2020 Authors: Gerhard Botha & Dr. Jan -Hendrik Keet PROPOSED EXPANSION OF THE SAND MINE AREA ON PORTION4 OF THE FARM ZANDBERG FONTEIN 97, SOUTH OF ROBERTSON, WESTERN CAPE PROVINCE Report Title: Botanical Study and Assessment Authors: Mr. Gerhard Botha and Dr. Jan-Hendrik Keet Project Name: Proposed expansion of the sand mine area on Portion 4 of the far Zandberg Fontein 97 south of Robertson, Western Cape Province Status of report: Version 1.0 Date: 6th April 2020 Prepared for: Greenmined Environmental Postnet Suite 62, Private Bag X15 Somerset West 7129 Cell: 082 734 5113 Email: [email protected] Prepared by Nkurenkuru Ecology and Biodiversity 3 Jock Meiring Street Park West Bloemfontein 9301 Cell: 083 412 1705 Email: gabotha11@gmail com Suggested report citation Nkurenkuru Ecology and Biodiversity, 2020. Section 102 Application (Expansion of mining footprint) and Final Basic Assessment & Environmental Management Plan for the proposed expansion of the sand mine on Portion 4 of the Farm Zandberg Fontein 97, Western Cape Province. Botanical Study and Assessment Report. Unpublished report prepared by Nkurenkuru Ecology and Biodiversity for GreenMined Environmental. Version 1.0, 6 April 2020. Proposed expansion of the zandberg sand mine April 2020 botanical STUDY AND ASSESSMENT I. DECLARATION OF CONSULTANTS INDEPENDENCE » act/ed as the independent specialist in this application; » regard the information contained in this -
Thesis Sci 2009 Bergh N G.Pdf
The copyright of this thesis vests in the author. No quotation from it or information derived from it is to be published without full acknowledgementTown of the source. The thesis is to be used for private study or non- commercial research purposes only. Cape Published by the University ofof Cape Town (UCT) in terms of the non-exclusive license granted to UCT by the author. University Systematics of the Relhaniinae (Asteraceae- Gnaphalieae) in southern Africa: geography and evolution in an endemic Cape plant lineage. Nicola Georgina Bergh Town Thesis presented for theCape Degree of DOCTOR OF ofPHILOSOPHY in the Department of Botany UNIVERSITY OF CAPE TOWN University May 2009 Town Cape of University ii ABSTRACT The Greater Cape Floristic Region (GCFR) houses a flora unique for its diversity and high endemicity. A large amount of the diversity is housed in just a few lineages, presumed to have radiated in the region. For many of these lineages there is no robust phylogenetic hypothesis of relationships, and few Cape plants have been examined for the spatial distribution of their population genetic variation. Such studies are especially relevant for the Cape where high rates of species diversification and the ongoing maintenance of species proliferation is hypothesised. Subtribe Relhaniinae of the daisy tribe Gnaphalieae is one such little-studied lineage. The taxonomic circumscription of this subtribe, the biogeography of its early diversification and its relationships to other members of the Gnaphalieae are elucidated by means of a dated phylogenetic hypothesis. Molecular DNA sequence data from both chloroplast and nuclear genomes are used to reconstruct evolutionary history using parsimony and Bayesian tools for phylogeny estimation. -
Vicariance, Climate Change, Anatomy and Phylogeny of Restionaceae
Botanical Journal of the Linnean Society (2000), 134: 159–177. With 12 figures doi:10.1006/bojl.2000.0368, available online at http://www.idealibrary.com on Under the microscope: plant anatomy and systematics. Edited by P. J. Rudall and P. Gasson Vicariance, climate change, anatomy and phylogeny of Restionaceae H. P. LINDER FLS Bolus Herbarium, University of Cape Town, Rondebosch 7701, South Africa Cutler suggested almost 30 years ago that there was convergent evolution between African and Australian Restionaceae in the distinctive culm anatomical features of Restionaceae. This was based on his interpretation of the homologies of the anatomical features, and these are here tested against a ‘supertree’ phylogeny, based on three separate phylogenies. The first is based on morphology and includes all genera; the other two are based on molecular sequences from the chloroplast genome; one covers the African genera, and the other the Australian genera. This analysis corroborates Cutler’s interpretation of convergent evolution between African and Australian Restionaceae. However, it indicates that for the Australian genera, the evolutionary pathway of the culm anatomy is much more complex than originally thought. In the most likely scenario, the ancestral Restionaceae have protective cells derived from the chlorenchyma. These persist in African Restionaceae, but are soon lost in Australian Restionaceae. Pillar cells and sclerenchyma ribs evolve early in the diversification of Australian Restionaceae, but are secondarily lost numerous times. In some of the reduction cases, the result is a very simple culm anatomy, which Cutler had interpreted as a primitively simple culm type, while in other cases it appears as if the functions of the ribs and pillars may have been taken over by a new structure, protective cells developed from epidermal, rather than chlorenchyma, cells. -
Plethora of Plants - Collections of the Botanical Garden, Faculty of Science, University of Zagreb (2): Glasshouse Succulents
NAT. CROAT. VOL. 27 No 2 407-420* ZAGREB December 31, 2018 professional paper/stručni članak – museum collections/muzejske zbirke DOI 10.20302/NC.2018.27.28 PLETHORA OF PLANTS - COLLECTIONS OF THE BOTANICAL GARDEN, FACULTY OF SCIENCE, UNIVERSITY OF ZAGREB (2): GLASSHOUSE SUCCULENTS Dubravka Sandev, Darko Mihelj & Sanja Kovačić Botanical Garden, Department of Biology, Faculty of Science, University of Zagreb, Marulićev trg 9a, HR-10000 Zagreb, Croatia (e-mail: [email protected]) Sandev, D., Mihelj, D. & Kovačić, S.: Plethora of plants – collections of the Botanical Garden, Faculty of Science, University of Zagreb (2): Glasshouse succulents. Nat. Croat. Vol. 27, No. 2, 407- 420*, 2018, Zagreb. In this paper, the plant lists of glasshouse succulents grown in the Botanical Garden from 1895 to 2017 are studied. Synonymy, nomenclature and origin of plant material were sorted. The lists of species grown in the last 122 years are constructed in such a way as to show that throughout that period at least 1423 taxa of succulent plants from 254 genera and 17 families inhabited the Garden’s cold glass- house collection. Key words: Zagreb Botanical Garden, Faculty of Science, historic plant collections, succulent col- lection Sandev, D., Mihelj, D. & Kovačić, S.: Obilje bilja – zbirke Botaničkoga vrta Prirodoslovno- matematičkog fakulteta Sveučilišta u Zagrebu (2): Stakleničke mesnatice. Nat. Croat. Vol. 27, No. 2, 407-420*, 2018, Zagreb. U ovom članku sastavljeni su popisi stakleničkih mesnatica uzgajanih u Botaničkom vrtu zagrebačkog Prirodoslovno-matematičkog fakulteta između 1895. i 2017. Uređena je sinonimka i no- menklatura te istraženo podrijetlo biljnog materijala. Rezultati pokazuju kako je tijekom 122 godine kroz zbirku mesnatica hladnog staklenika prošlo najmanje 1423 svojti iz 254 rodova i 17 porodica. -
Pollination Structures Plant and Nectar‐Feeding Bird
Received: 10 April 2020 Revised: 22 April 2020 Accepted: 3 May 2020 DOI: 10.1111/1440-1703.12148 ORIGINAL ARTICLE Pollination structures plant and nectar-feeding bird communities in Cape fynbos, South Africa: Implications for the conservation of plant–bird mutualisms Sjirk Geerts1 | Anina Coetzee2 | Anthony G. Rebelo3 | Anton Pauw4 1Department Conservation and Marine Sciences, Cape Peninsula University of Abstract Technology, Cape Town, South Africa With the current global concerns about pollinators, relationships between spe- 2DST/NRF Centre of Excellence at the cies interactions and diversity are pivotal. If pollinator communities depend FitzPatrick Institute of African strongly on the diversity of flowering plants and vice versa, anthropogenic Ornithology, University of Cape Town, Cape Town, South Africa influences—whether positive or negative—on one partner will cause changes 3South African National Biodiversity in the other. Here we ask whether nectarivorous bird communities are struc- Institute, Kirstenbosch Research Centre, tured by resource abundance (Proteaceae nectar) or Proteaceae diversity at dif- Claremont, South Africa ferent spatial scales in the Cape fynbos of South Africa. On a small spatial 4Department of Botany and Zoology, Stellenbosch University, Matieland, scale, we sampled 34 one-hectare plots across the Cape Floristic Region (CFR) South Africa for flowering Proteaceae species, number of inflorescences, nectar volume, veg- etation age, nectar-feeding bird abundance and species richness. At small Correspondence Sjirk Geerts, Department Conservation scale, nectar—rather than vegetation structure or plant community and Marine Sciences, Cape Peninsula composition—was the most strongly correlated to nectar-feeding bird diversity University of Technology, P.O. Box and abundance. On a landscape scale we investigated the spatio-temporal 652, Cape Town 8000, South Africa. -
Field Guide for Wild Flower Harvesting
FIELD GUIDE FOR WILD FLOWER HARVESTING 1 Contents Introducing the Field Guide for Wild Flower Harvesting 3 Glossary 4 Introducing The Field Guide Fynbos 6 for Wild Flower Harvesting What is fynbos? 7 The Cape Floral Kingdom 7 Many people in the Overberg earn a living from the region’s wild flowers, known as South African plants 8 fynbos. Some pick flowers for markets to sell, some remove invasive alien plants, and Threats to fynbos 8 others are involved in conservation and nature tourism. It is important that people The value of fynbos 9 who work in the veld know about fynbos plants. This Field Guide for Wild Flower Harvesting describes 41 of the most popular types of fynbos plants that are picked from Fynbos and fire 9 our region for the wild flower market. It also provides useful information to support Classification of plants 9 sustainable harvesting in particular and fynbos conservation in general. Naming of plants 10 Picking flowers has an effect or impact on the veld. If we are not careful, we can Market for fynbos 10 damage, or even kill, plants. So, before picking flowers, it is important to ask: Picking fynbos with care 11 • What can be picked? The Sustainable Harvesting Programme 12 • How much can be picked? • How should flowers be picked? The SHP Code of Best Practice for Wild Harvesters 12 Ten principles of good harvesting 13 This guide aims to help people understand: The Vulnerability Index and the Red Data List 13 • the differences between the many types of fynbos plants that grow in the veld; and Know how much fynbos you have 14 • which fynbos plants can be picked, and which are scarce and should rather be Fynbos plants of the Agulhas Plain and beyond 14 left in the veld.