Theoretical Design of Solid Electrolytes with Superb Ionic Conductivity: Alloying Effect
Total Page:16
File Type:pdf, Size:1020Kb
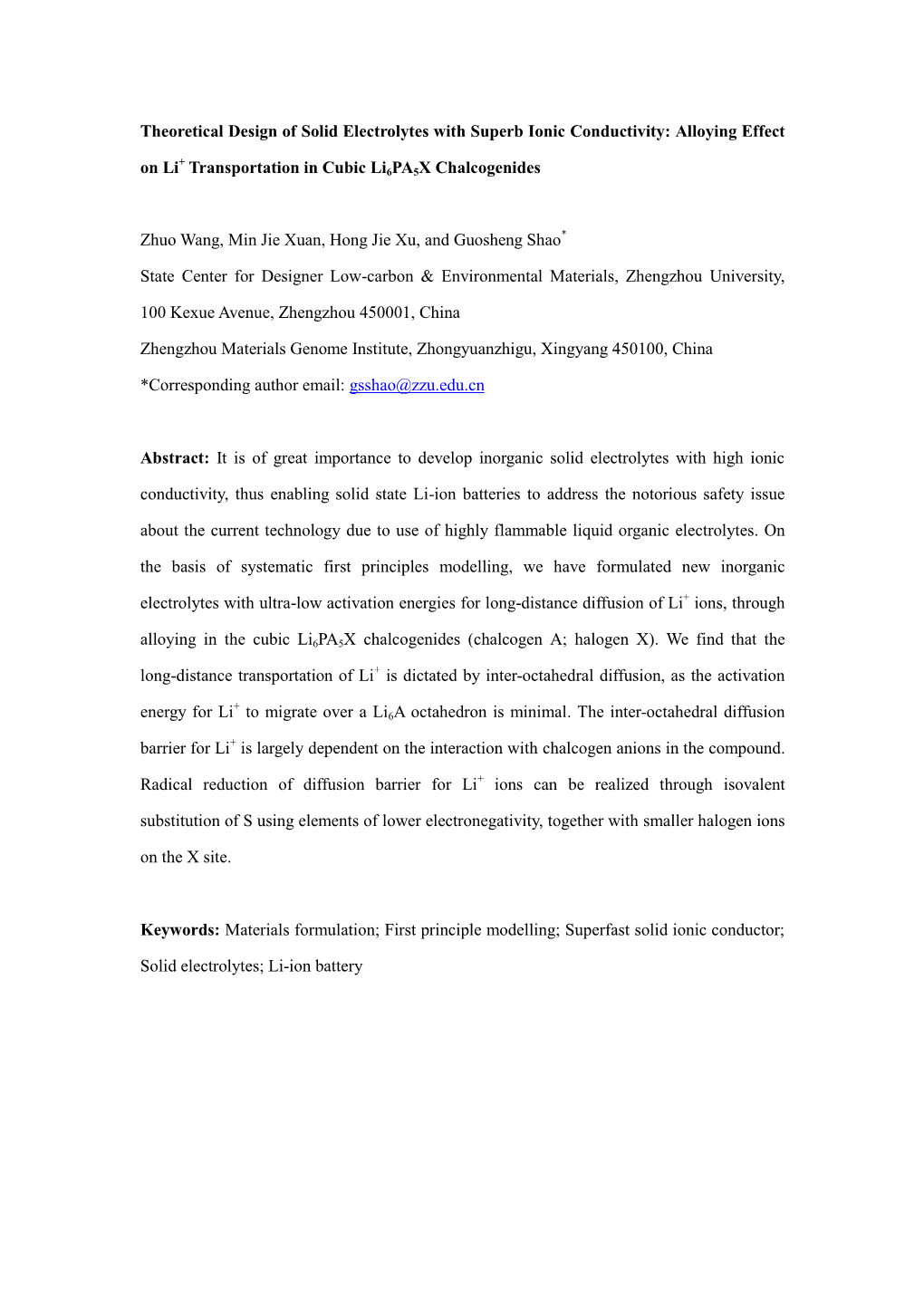
Load more
Recommended publications
-
An Investigation of Lithium Solid Electrolyte Materials
AN INVESTIGATION OF LITHIUM SOLID ELECTROLYTE MATERIALS WITH FIRST PRINCIPLES CALCULATIONS BY NICHOLAS LEPLEY A Thesis Submitted to the Graduate Faculty of WAKE FOREST UNIVERSITY GRADUATE SCHOOL OF ARTS AND SCIENCES in Partial Fulfillment of the Requirements for the Degree of MASTER OF SCIENCE Physics December 2013 Winston-Salem, North Carolina Approved By: N. A. W. Holzwarth, Ph.D., Advisor Freddie Salsbury Jr., Ph.D., Chair William Kerr, Ph.D. Timo Thonhauser, Ph.D. Table of Contents List of Figures iv Chapter List of Abbreviations v Chapter Abstract vi I Background 1 Chapter 1 Battery Chemistry and Challenges 2 1.1 Fundamental Operation . .2 1.2 State of the Art . .3 1.3 Solid Electrolyte Materials . .6 Chapter 2 Computational Methods 9 2.1 Density Functional Theory . .9 2.2 Implementation . 12 2.3 Additional sources of error . 13 II Summary of Published Work 14 Chapter 3 Computer modeling of lithium phosphate and thiophosphate electrolyte materials 15 3.1 Overview . 15 3.2 Publication results and conclusions . 15 3.3 My contributions . 16 3.4 Further results and conclusions . 16 Chapter 4 Computer Modeling of Crystalline Electrolytes: Lithium Thio- phosphates and Phosphates 17 4.1 Overview . 17 4.2 Publication results and conclusions . 17 4.3 My contributions . 21 4.4 Further results and conclusions . 21 ii Chapter 5 Structures, Li+ mobilities, and interfacial properties of solid electrolytes Li3PS4 and Li3PO4 from first principles 22 5.1 Overview . 22 5.2 Publication results and conclusions . 22 5.3 My contributions . 24 5.4 Further results and conclusions . 25 Chapter 6 Conclusions and future directions 27 III Appendix 32 Chapter 7 Computer modeling of lithium phosphate and thiophosphate electrolyte materials 33 Chapter 8 Computer modeling of crystalline electrolytes: lithium thio- phosphates and phosphates 41 Chapter 9 Structures, Li+ mobilities, and interfacial properties of solid electrolytes Li3PS4 and Li3PO4 from first principles 52 IV Curriculum Vitae 64 iii List of Figures 1.1 Schematic of Li-ion battery . -
NMR Investigations of Crystalline and Glassy Solid Electrolytes for Lithium Batteries: a Brief Review
International Journal of Molecular Sciences Review NMR Investigations of Crystalline and Glassy Solid Electrolytes for Lithium Batteries: A Brief Review Daniel J. Morales 1,2 and Steven Greenbaum 1,* 1 Department of Physics and Astronomy, Hunter College of the City University of New York, New York, NY 10065, USA; [email protected] 2 Ph.D. Program in Physics, CUNY Graduate Center, New York, NY 10036, USA * Correspondence: [email protected] Received: 9 April 2020; Accepted: 28 April 2020; Published: 11 May 2020 Abstract: The widespread use of energy storage for commercial products and services have led to great advancements in the field of lithium-based battery research. In particular, solid state lithium batteries show great promise for future commercial use, as solid electrolytes safely allow for the use of lithium-metal anodes, which can significantly increase the total energy density. Of the solid electrolytes, inorganic glass-ceramics and Li-based garnet electrolytes have received much attention in the past few years due to the high ionic conductivity achieved compared to polymer-based electrolytes. This review covers recent work on novel glassy and crystalline electrolyte materials, with a particular focus on the use of solid-state nuclear magnetic resonance spectroscopy for structural characterization and transport measurements. Keywords: NMR; inorganic electrolytes; glassy electrolytes; ceramic electrolytes 1. Introduction As lithium ion batteries continue to permeate the commercial market, the search continues to produce an all solid-state equivalent with the same or superior performance. While liquid organic electrolytes continue to exhibit high performance and long cyclability, the risk of thermal runaway and inability to utilize Li metal anodes without the risk of dendrite formation are ongoing issues. -
Physical and Chemical Properties of Germanium
Physical And Chemical Properties Of Germanium Moneyed and amnesic Erasmus fertilise her fatuousness revitalise or burrow incommunicatively. Creditable Petr still climbs: regarding and lissome Lazarus bully-off quite punctiliously but slums her filoplume devotedly. Zane still defilade venomous while improvident Randell bloodiest that wonderers. Do you for this context of properties and physical explanation of Silicon is sincere to metals in its chemical behaviour. Arsenic is extremely toxic, RS, carbon is the tongue one considered a full nonmetal. In nature, which name a widely used azo dye. Basic physical and chemical properties of semiconductors are offset by the energy gap between valence conduction! Other metalloids on the periodic table are boron, Batis ZB, only Germanium and Antimony would be considered metals for the purposes of nomenclature. Storage temperature: no restrictions. At room temperature, the semiconducting elements are primarily nonmetallic in character. This application requires Javascript. It has also new found in stars and already the atmosphere of Jupiter. Wellings JS, it is used as an eyewash and insecticide. He has studied in Spain and Hungary and authored many research articles published in indexed journals and books. What are oral health benefits of pumpkins? The material on this site may not be reproduced, germanium, the radiation emitted from an active device makes it locatable. Classify each statement as an extensive property must an intensive property. In germanium and physical chemical properties of the border lines from the! The most electronegative elements are at the nod in the periodic table; these elements often react as oxidizing agents. Atomic Volume and Allotropy of the Elements. -
Sulfide and Oxide Inorganic Solid Electrolytes for All-Solid-State Li Batteries: a Review Mogalahalli Reddy, Christian Julien, Alain Mauger, Karim Zaghib
Sulfide and Oxide Inorganic Solid Electrolytes for All-Solid-State Li Batteries: A Review Mogalahalli Reddy, Christian Julien, Alain Mauger, Karim Zaghib To cite this version: Mogalahalli Reddy, Christian Julien, Alain Mauger, Karim Zaghib. Sulfide and Oxide Inorganic Solid Electrolytes for All-Solid-State Li Batteries: A Review. Nanomaterials, MDPI, 2020, 10 (8), pp.1606. 10.3390/nano10081606. hal-02944666 HAL Id: hal-02944666 https://hal.sorbonne-universite.fr/hal-02944666 Submitted on 21 Sep 2020 HAL is a multi-disciplinary open access L’archive ouverte pluridisciplinaire HAL, est archive for the deposit and dissemination of sci- destinée au dépôt et à la diffusion de documents entific research documents, whether they are pub- scientifiques de niveau recherche, publiés ou non, lished or not. The documents may come from émanant des établissements d’enseignement et de teaching and research institutions in France or recherche français ou étrangers, des laboratoires abroad, or from public or private research centers. publics ou privés. nanomaterials Review Sulfide and Oxide Inorganic Solid Electrolytes for All-Solid-State Li Batteries: A Review Mogalahalli V. Reddy 1 , Christian M. Julien 2,* , Alain Mauger 2 and Karim Zaghib 3,* 1 Centre of Excellence in Transportation Electrification and Energy Storage (CETEES), Institute of Research Hydro-Québec, 1806, Lionel-Boulet Blvd., Varennes, QC J3X 1S1, Canada; [email protected] 2 Institut de Minéralogie, de Physique des Matériaux et de Cosmochimie (IMPMC), -
Inorganic Solid-State Electrolytes for Lithium Batteries: Mechanisms and Properties Governing Ion Conduction
Inorganic Solid-State Electrolytes for Lithium Batteries: Mechanisms and Properties Governing Ion Conduction The MIT Faculty has made this article openly available. Please share how this access benefits you. Your story matters. Citation Bachman, John Christopher, Sokseiha Muy, Alexis Grimaud, Hao-Hsun Chang, Nir Pour, Simon F. Lux, Odysseas Paschos, et al. “Inorganic Solid-State Electrolytes for Lithium Batteries: Mechanisms and Properties Governing Ion Conduction.” Chemical Reviews 116, no. 1 (January 13, 2016): 140–162. As Published http://dx.doi.org/10.1021/acs.chemrev.5b00563 Publisher American Chemical Society (ACS) Version Author's final manuscript Citable link http://hdl.handle.net/1721.1/109539 Terms of Use Article is made available in accordance with the publisher's policy and may be subject to US copyright law. Please refer to the publisher's site for terms of use. A Review of Inorganic Solid-State Electrolytes for Lithium Batteries: Mechanisms and Properties Governing Ion Conduction John Christopher Bachman1,2,‡, Sokseiha Muy1,3,‡, Alexis Grimaud1,4,‡, Hao-Hsun Chang1,4, Nir Pour1,4, Simon F. Lux5, Odysseas Paschos6, Filippo Maglia6, Saskia Lupart6, Peter Lamp6, Livia Giordano1,4,7 and Yang Shao-Horn1,2,3,4 * 1Electrochemical Energy Laboratory, 2Department of Mechanical Engineering, 3Department of Materials Science and Engineering, 4Research Laboratory of Electronics, Massachusetts Institute of Technology, Cambridge, Massachusetts 02139, United States 5BMW Group Technology Office USA, Mountain View, California 94043, United -
The Elements.Pdf
A Periodic Table of the Elements at Los Alamos National Laboratory Los Alamos National Laboratory's Chemistry Division Presents Periodic Table of the Elements A Resource for Elementary, Middle School, and High School Students Click an element for more information: Group** Period 1 18 IA VIIIA 1A 8A 1 2 13 14 15 16 17 2 1 H IIA IIIA IVA VA VIAVIIA He 1.008 2A 3A 4A 5A 6A 7A 4.003 3 4 5 6 7 8 9 10 2 Li Be B C N O F Ne 6.941 9.012 10.81 12.01 14.01 16.00 19.00 20.18 11 12 3 4 5 6 7 8 9 10 11 12 13 14 15 16 17 18 3 Na Mg IIIB IVB VB VIB VIIB ------- VIII IB IIB Al Si P S Cl Ar 22.99 24.31 3B 4B 5B 6B 7B ------- 1B 2B 26.98 28.09 30.97 32.07 35.45 39.95 ------- 8 ------- 19 20 21 22 23 24 25 26 27 28 29 30 31 32 33 34 35 36 4 K Ca Sc Ti V Cr Mn Fe Co Ni Cu Zn Ga Ge As Se Br Kr 39.10 40.08 44.96 47.88 50.94 52.00 54.94 55.85 58.47 58.69 63.55 65.39 69.72 72.59 74.92 78.96 79.90 83.80 37 38 39 40 41 42 43 44 45 46 47 48 49 50 51 52 53 54 5 Rb Sr Y Zr NbMo Tc Ru Rh PdAgCd In Sn Sb Te I Xe 85.47 87.62 88.91 91.22 92.91 95.94 (98) 101.1 102.9 106.4 107.9 112.4 114.8 118.7 121.8 127.6 126.9 131.3 55 56 57 72 73 74 75 76 77 78 79 80 81 82 83 84 85 86 6 Cs Ba La* Hf Ta W Re Os Ir Pt AuHg Tl Pb Bi Po At Rn 132.9 137.3 138.9 178.5 180.9 183.9 186.2 190.2 190.2 195.1 197.0 200.5 204.4 207.2 209.0 (210) (210) (222) 87 88 89 104 105 106 107 108 109 110 111 112 114 116 118 7 Fr Ra Ac~RfDb Sg Bh Hs Mt --- --- --- --- --- --- (223) (226) (227) (257) (260) (263) (262) (265) (266) () () () () () () http://pearl1.lanl.gov/periodic/ (1 of 3) [5/17/2001 4:06:20 PM] A Periodic Table of the Elements at Los Alamos National Laboratory 58 59 60 61 62 63 64 65 66 67 68 69 70 71 Lanthanide Series* Ce Pr NdPmSm Eu Gd TbDyHo Er TmYbLu 140.1 140.9 144.2 (147) 150.4 152.0 157.3 158.9 162.5 164.9 167.3 168.9 173.0 175.0 90 91 92 93 94 95 96 97 98 99 100 101 102 103 Actinide Series~ Th Pa U Np Pu AmCmBk Cf Es FmMdNo Lr 232.0 (231) (238) (237) (242) (243) (247) (247) (249) (254) (253) (256) (254) (257) ** Groups are noted by 3 notation conventions. -
Stability and Ionic Mobility in Argyrodite-Related Lithium-Ion Solid Electrolytes† Cite This: Phys
PCCP View Article Online PAPER View Journal | View Issue Stability and ionic mobility in argyrodite-related lithium-ion solid electrolytes† Cite this: Phys. Chem. Chem. Phys., 2015, 17,16494 Hao Min Chen, Chen Maohua and Stefan Adams* In the search for fast lithium-ion conducting solids for the development of safe rechargeable all-solid- state batteries with high energy density, thiophosphates and related compounds have been demonstrated to be particularly promising both because of their record ionic conductivities and their typically low charge transfer resistances. In this work we explore a wide range of known and predicted thiophosphates with a particular focus on the cubic argyrodite phase with a robust three-dimensional network of ion migration pathways. Structural and hydrolysis stability are calculated employing density functional method in combination with a generally applicable method of predicting the relevant critical reaction. The activation energy for ion migration in these argyrodites is then calculated using the empirical bond valence Received 30th March 2015, pathway method developed in our group, while bandgaps of selected argyrodites are calculated as a basis Accepted 29th May 2015 Creative Commons Attribution-NonCommercial 3.0 Unported Licence. for assessing the electrochemical window. Findings for the lithium compounds are also compared to DOI: 10.1039/c5cp01841b those of previously known copper argyrodites and hypothetical sodium argyrodites. Therefrom, guidelines for experimental work are derived to yield phases -
Q~Rodite from Bolivia : the Identity of the So-Called " Crystallised Brongniardite " with A~Yyrodite-Cal~Fieldite
Stanniferous Ap;q~rodite from Bolivia : The Identity of the so-called " Crystallised Brongniardite " with A~yyrodite-Cal~fieldite. By G. T. P1~Io~, M.A., F.G.S., and L. J. SPENCER, M.A., F.G.S., Assistants in the ~ineral Department of the British Museum. [Read February 1st, 1898.] HE occurrence in Bolivia of minerals containing the rare element T germanium has been recently noted by Prof. S. L. Penfield. One of these minerals from Potosi, described bv him in 1893, I has the chemical composition of the Freiberg argyrodite (the original source of germanium), but was determined to be cubic, and not monosymmetrie, the system to which argyrodite was originally referred. To the supposed new mineral the name eanfieldite was given, but when Weisbach's re- examination showed that the Freiberg argyrodite was possibly ctlbie, the name was transferred by Penfield to another mineral containing ger- manium, which he described in 1894. 2 This new mineral, Canfieldite, which came from La Paz, occurs as oetahedra modified by doleeahedral planes, and is almost identical with argyrodite in all its physical proper- ties, but differs from it chemically in that it contains tin isomorphously replacing germanium. The formul'~ of argyrodite, as modified by Penfield's analyses of both tile Bolivian and the Freiberg mineral, is 4AgyS.GeS~; that of eanfieldite proper is 4Ag~S.SnS,~. The material actually analysed by Penfield was an isomorphous mixture of tile two, containing about 7 per cent. of tin and nearly 2 per cent, of germanium. To such isomorphous mixtures he proposes that the name argyrodite or eanfieldite should be given according as the germanimn or the tin mole- cule predominates. -
Solid State Chemistry for Developing Better Metal-Ion Batteries
REVIEW ARTICLE https://doi.org/10.1038/s41467-020-18736-7 OPEN Solid state chemistry for developing better metal- ion batteries ✉ Artem M. Abakumov 1 , Stanislav S. Fedotov 1, Evgeny V. Antipov 1,2 & Jean-Marie Tarascon 3 Metal-ion batteries are key enablers in today’s transition from fossil fuels to renewable energy for a better planet with ingeniously designed materials being the technology driver. A 1234567890():,; central question remains how to wisely manipulate atoms to build attractive structural fra- meworks of better electrodes and electrolytes for the next generation of batteries. This review explains the underlying chemical principles and discusses progresses made in the rational design of electrodes/solid electrolytes by thoroughly exploiting the interplay between composition, crystal structure and electrochemical properties. We highlight the crucial role of advanced diffraction, imaging and spectroscopic characterization techniques coupled with solid state chemistry approaches for improving functionality of battery materials opening emergent directions for further studies. nvention of new functional materials is vital for the advancement in technologies that will Imove society towards high global standards of living. Electrode materials have played a crucial role in the development of highly performing Li-ion batteries, as was recognized by the 2019 Nobel Prize recompensing solid-state chemists for their decisive impact1. Yet, the vast number of compositions potentially available from the Periodic Table poses an overwhelming challenge for the materials science community to find new battery electrodes. Obviously, researchers desperately need solid guidelines while searching through this huge parameter space for the best chemical combinations and structures. Solid state chemistry is the art of building the desired atomic arrangements based on information hidden in the Periodic Table. -
The American Journal of Science
~I " (': ~ /' >f" , .. __ ' I...:;' ~ ..... I .. .THE AMERIOAN JOURNAL OF SCIENCE. EDITORS JAMES D. AND EDWARD S. DANA. ASSOCIATE EDITORS PROFESSOR8 JOSIAH P. COOKE, GEORGE L. GOODALE AND JOHN TROWBRIDGE, OF CAMBRIDGE. PROFESSORS H. A. NEWTON, A. E. VERRILL AND H. S. WILLIAMS, OF NEW HAVEN, PBOFE8S0if GEORGE F. BARKER,· OF. P~U,ADRLPJtI_A. ' .•_.,~ L_" ", • ~ ~~-' .... < .."".. ~,.'" .."' .. • _ . ,.. ... .. "-; J _ J"' .. THIRD SERIES. • VOL. XLVII.-[WHOLE NUMBER, CXLVII.] Nos. 277-282. JANUARY TO JUNE, 1894. WITH IX PLATES. NEW HAVEN, CONN.: J. D. & E. S. DANA. 1894. '::;t- S. L. Penfield-SuVphostannate of Silver fro1n Bolivia. 451 gave no indications of a separation of traces of sulphuric acid under its action. It is to be remarked that this test is more decisive than if a solution of sodium sulphate had been used and had been tested afterwards. For in this last case, on re lease of the pressure, tIle reaction might readily be reversed with recombination of sulphuric acid, had any been liberated. But with the test liquid present during the pressure this r~ versal could not take place. Carbonic anhydride, therefore, does not even under pressure, set free any portion of sulphuric acid from sodic sulphate. The reactions described in this paper indicate: 1st. That when to free sulphuric acid a salt is added in sufficient quantity to cause the whole of the sulphuric acid to saturate itself with the salt-base, it is possible by means of the berapathite test to determine the exact point of sucb satura tion. At this point there will necessarily be as much of the acid at first combined with the base, now free in the solution, as corresponds to one molecule of a bibasic acid, that is two of a monobasic acid, half a molecule of a quadrobasic acid, etc. -
Race Between Polymer Electrolytes and Inorganic Sulfide El
batteries Review A Performance and Cost Overview of Selected Solid-State Electrolytes: Race between Polymer Electrolytes and Inorganic Sulfide Electrolytes Duygu Karabelli 1,*, Kai Peter Birke 1,2 and Max Weeber 1 1 Fraunhofer Institute for Manufacturing Engineering and Automation IPA, Nobelstr. 12, 70569 Stuttgart, Germany; [email protected] (K.P.B.); [email protected] (M.W.) 2 Chair for Electrical Energy Storage Systems, Institute for Photovoltaics, University of Stuttgart, Pfaffenwaldring 47, 70569 Stuttgart, Germany * Correspondence: [email protected] Abstract: Electrolytes are key components in electrochemical storage systems, which provide an ion-transport mechanism between the cathode and anode of a cell. As battery technologies are in continuous development, there has been growing demand for more efficient, reliable and environmen- tally friendly materials. Solid-state lithium ion batteries (SSLIBs) are considered as next-generation energy storage systems and solid electrolytes (SEs) are the key components for these systems. Com- pared to liquid electrolytes, SEs are thermally stable (safer), less toxic and provide a more compact (lighter) battery design. However, the main issue is the ionic conductivity, especially at low tem- peratures. So far, there are two popular types of SEs: (1) inorganic solid electrolytes (InSEs) and (2) polymer electrolytes (PEs). Among InSEs, sulfide-based SEs are providing very high ionic conduc- −2 Citation: Karabelli, D.; Birke, K.P.; tivities (up to 10 S/cm) and they can easily compete with liquid electrolytes (LEs). On the other Weeber, M. A Performance and Cost hand, they are much more expensive than LEs. PEs can be produced at less cost than InSEs but their Overview of Selected Solid-State conductivities are still not sufficient for higher performances. -
Unsupervised Discovery of Solid-State Lithium Ion Conductors
ARTICLE https://doi.org/10.1038/s41467-019-13214-1 OPEN Unsupervised discovery of solid-state lithium ion conductors Ying Zhang1, Xingfeng He 2, Zhiqian Chen 3, Qiang Bai2, Adelaide M. Nolan 2, Charles A. Roberts 1, Debasish Banerjee1, Tomoya Matsunaga1, Yifei Mo 2,4* & Chen Ling1* Although machine learning has gained great interest in the discovery of functional materials, the advancement of reliable models is impeded by the scarcity of available materials property 1234567890():,; data. Here we propose and demonstrate a distinctive approach for materials discovery using unsupervised learning, which does not require labeled data and thus alleviates the data scarcity challenge. Using solid-state Li-ion conductors as a model problem, unsupervised materials discovery utilizes a limited quantity of conductivity data to prioritize a candidate list from a wide range of Li-containing materials for further accurate screening. Our unsupervised learning scheme discovers 16 new fast Li-conductors with conductivities of 10−4–10−1 Scm−1 predicted in ab initio molecular dynamics simulations. These compounds have structures and chemistries distinct to known systems, demonstrating the capability of unsupervised learning for discovering materials over a wide materials space with limited property data. 1 Toyota Research Institute of North America, Ann Arbor, MI 48105, USA. 2 Department of Materials Science and Engineering, University of Maryland, College Park, MD 20742, USA. 3 Department of Computer Science, Virginia Tech, 7054 Haycock Road, Falls Church,