Posttranslational Modification of Waxy to Genetically Improve Starch
Total Page:16
File Type:pdf, Size:1020Kb
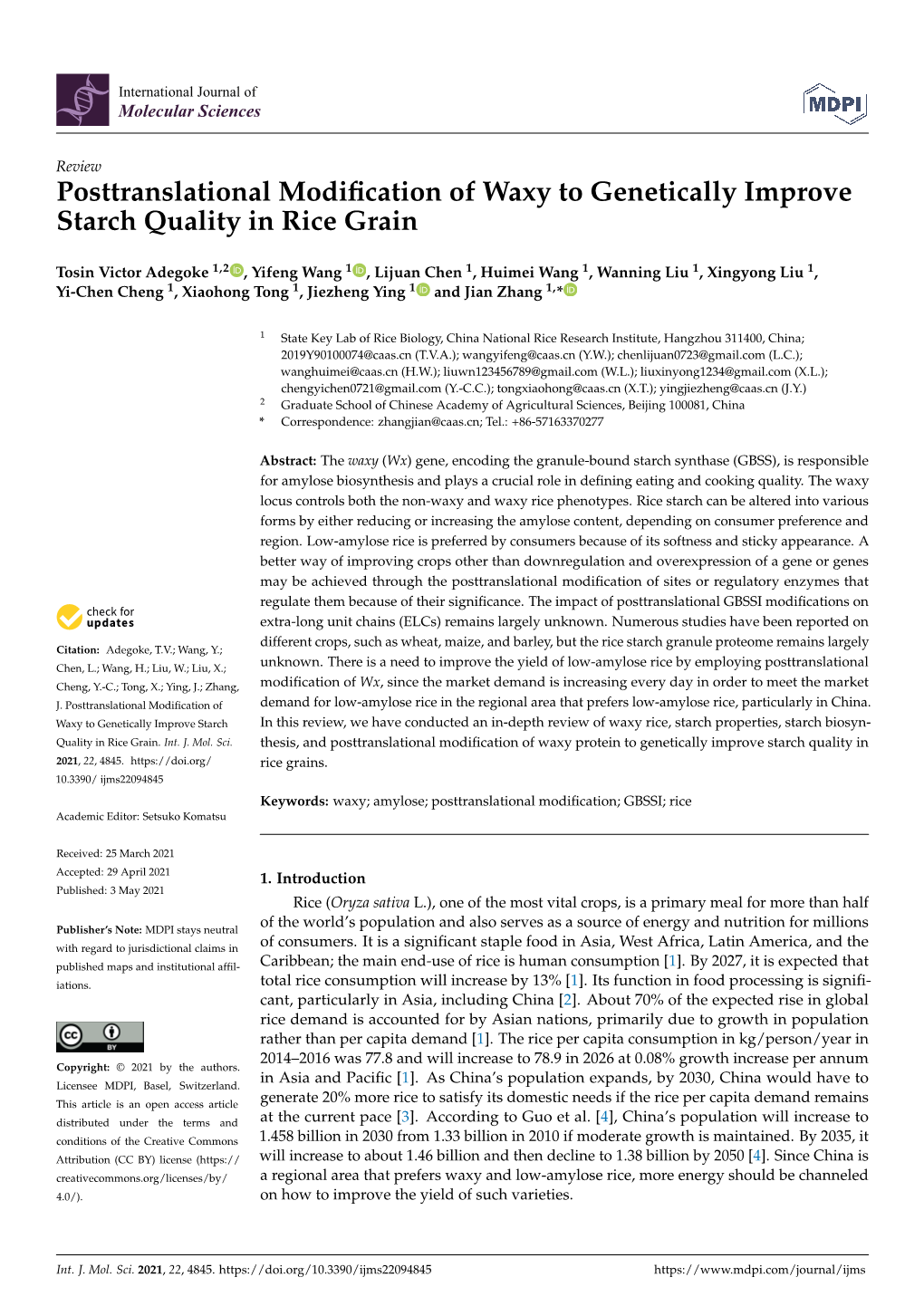
Load more
Recommended publications
-
Root Hydrotropism, Or Any Other Plant Differential C
American Journal of Botany 100(1): 14–24. 2013. R OOT HYDROTROPISM: AN UPDATE 1 G LADYS I. CASSAB 2 , D ELFEENA E APEN, AND M ARÍA EUGENIA C AMPOS Departamento de Biología Molecular de Plantas, Instituto de Biotecnología, Universidad Nacional Autónoma de México, Apdo. Postal 510-3, Col. Chamilpa, Cuernavaca, Mor. 62250 México While water shortage remains the single-most important factor infl uencing world agriculture, there are very few studies on how plants grow in response to water potential, i.e., hydrotropism. Terrestrial plant roots dwell in the soil, and their ability to grow and explore underground requires many sensors for stimuli such as gravity, humidity gradients, light, mechanical stimulations, tem- perature, and oxygen. To date, extremely limited information is available on the components of such sensors; however, all of these stimuli are sensed in the root cap. Directional growth of roots is controlled by gravity, which is fi xed in direction and intensity. However, other environmental factors, such as water potential gradients, which fl uctuate in time, space, direction, and intensity, can act as a signal for modifying the direction of root growth accordingly. Hydrotropism may help roots to obtain water from the soil and at the same time may participate in the establishment of the root system. Current genetic analysis of hydrotropism in Arabidopsis has offered new players, mainly AHR1, NHR1, MIZ1 , and MIZ2 , which seem to modulate how root caps sense and choose to respond hydrotropically as opposed to other tropic responses. Here we review the mechanism(s) by which these genes and the plant hormones abscisic acid and cytokinins coordinate hydrotropism to counteract the tropic responses to gravitational fi eld, light or touch stimuli. -
Plant:Animal Cell Comparison
Comparing Plant And Animal Cells http://khanacademy.org/video?v=Hmwvj9X4GNY Plant Cells shape - most plant cells are squarish or rectangular in shape. amyloplast (starch storage organelle)- an organelle in some plant cells that stores starch. Amyloplasts are found in starchy plants like tubers and fruits. cell membrane - the thin layer of protein and fat that surrounds the cell, but is inside the cell wall. The cell membrane is semipermeable, allowing some substances to pass into the cell and blocking others. cell wall - a thick, rigid membrane that surrounds a plant cell. This layer of cellulose fiber gives the cell most of its support and structure. The cell wall also bonds with other cell walls to form the structure of the plant. chloroplast - an elongated or disc-shaped organelle containing chlorophyll. Photosynthesis (in which energy from sunlight is converted into chemical energy - food) takes place in the chloroplasts. chlorophyll - chlorophyll is a molecule that can use light energy from sunlight to turn water and carbon dioxide gas into glucose and oxygen (i.e. photosynthesis). Chlorophyll is green. cytoplasm - the jellylike material outside the cell nucleus in which the organelles are located. Golgi body - (or the golgi apparatus or golgi complex) a flattened, layered, sac-like organelle that looks like a stack of pancakes and is located near the nucleus. The golgi body modifies, processes and packages proteins, lipids and carbohydrates into membrane-bound vesicles for "export" from the cell. lysosome - vesicles containing digestive enzymes. Where the digestion of cell nutrients takes place. mitochondrion - spherical to rod-shaped organelles with a double membrane. -
Ultrastructure of Cells and Microanalysis in Malus Domestica Borkh. 'Szampion' Fruit in Relation to Varied Calcium Foliar Fe
molecules Article Ultrastructure of Cells and Microanalysis in Malus domestica Borkh. ‘Szampion’ Fruit in Relation to Varied Calcium Foliar Feeding Piotr Kowalik 1, Tomasz Lipa 1 , Zenia Michałoj´c 1 and Mirosława Chwil 2,* 1 Institute of Horticulture Production, University of Life Sciences in Lublin, Akademicka 15, 20-950 Lublin, Poland; [email protected] (P.K.); [email protected] (T.L.); [email protected] (Z.M.) 2 Department of Botany and Plant Physiology, University of Life Sciences in Lublin, Akademicka 15, 20-950 Lublin, Poland * Correspondence: [email protected] Received: 18 August 2020; Accepted: 8 October 2020; Published: 11 October 2020 Abstract: Calcium is one of the most poorly reutilized nutrients. Its deficiencies cause various physiological disturbances and, consequently, reduce the quantity and quality of yields. Reduced content of Ca2+ ions in cells leads to development of, e.g., bitter pit in apples. Efficient and instantaneous mitigation of Ca2+ deficiencies is provided by foliar feeding. There are no detailed data on the effect of foliar feeding with various calcium forms on the cell structure or on the microanalysis and mapping of this element in apple fruit cells. Therefore, we carried out comparative studies of the ultrastructure of epidermis and hypodermis cells, to assess the content and distribution of calcium in the cell wall, cytoplasmic membrane, cytoplasm, and precipitates of Malus domestica Borkh. ‘Szampion’ fruit exposed to four Ca treatments, including the control with no additional Ca supplementation (I) and foliar applications of Ca(NO3)2 (II), CaCl2 (III), and Ca chelated with EDTA (IV). -
2 the Structure and Ultrastructure of the Cell Gunther Neuhaus Institut Fu¨R Zellbiologie, Freiburg, Germany
2 The Structure and Ultrastructure of the Cell Gunther Neuhaus Institut fu¨r Zellbiologie, Freiburg, Germany 2.1 Cell Biology . .........................40 2.2.7.6 Isolating Secondary Walls . 107 2.1.1 Light Microscopy . 43 2.2.8 Mitochondria . 109 2.1.2 Electron Microscopy . 45 2.2.8.1 Shape Dynamics and Reproduction . 110 2.2.8.2 Membranes and Compartmentalization in 2.2 The Plant Cell . .........................46 Mitochondria . 112 2.2.1 Overview . 46 2.2.9 Plastids . 113 2.2.2 The Cytoplasm . 50 2.2.9.1 Form and Ultrastructure of 2.2.2.1 The Cytoskeleton . 51 Chloroplasts . 114 2.2.2.2 Motor Proteins and Cellular Kinetic 2.2.9.2 Other Plastid Types, Starches . 116 Processes . 55 2.2.2.3 Flagella and Centrioles . 57 2.3 Cell Structure in Prokaryotes ............. 119 2.2.3 The Cell Nucleus . 59 2.3.1 Reproduction and Genetic Apparatus . 122 2.2.3.1 Chromatin . 60 2.3.2 Bacterial Flagella . 124 2.2.3.2 Chromosomes and Karyotype . 63 2.3.3 Wall Structures . 125 2.2.3.3 Nucleoli and Preribosomes . 64 2.2.3.4 Nuclear Matrix and Nuclear Membrane . 65 2.4 The Endosymbiotic Theory and the 2.2.3.5 Mitosis and the Cell Cycle . 66 Hydrogen Hypothesis . ............. 125 2.2.3.6 Cell Division . 73 2.4.1 Endocytobiosis . 126 2.2.3.7 Meiosis . 73 2.4.2 Origin of Plastids and Mitochondria by 2.2.3.8 Crossing-Over . 79 Symbiogenesis . 127 2.2.3.9 Syngamy . 79 2.2.4 Ribosomes . -
Identifying Genetic Factors That Contribute to Trait Variation Among Species Is Difficult
Identifying genetic factors that contribute to trait variation among species is difficult. Weiss et al. developed a method for unbiased genome-scale mapping of trait differences and used it to work out how a range of yeast species evolved. When crossed, these yeast species produce sterile offspring, preventing the use of traditional genetic-mapping techniques. However, by reciprocal hemizygosity analysis via sequencing (RH-seq), alleles that contributed to differences in thermotolerance were spotted that diverged between two species. Surprisingly, these turned out to map to essential housekeeping genes. Thus, without taking a candidate locus approach, RH-seq allowed identification of genes responsible for traits that differ between species. Nat. Genet. 50, 1501 (2018). https://www.sciencemag.org/news/2018/11/researchers-launch-plan-sequence-66000-species-united-kingdom-s-just- start?utm_campaign=news_weekly_2018-11-02&et_rid=328058488&et_cid=2465523 The Earth BioGenome Project aims to sequence the genome of every complex organism on Earth — that’s 1.5 million species — and will probably cost $US4.7 billion. The project brings together more than a dozen existing ventures that focus on various slices of life, such as specific types of animal or the creatures of a particular country. Among the largest commitments to the effort so far is a plan by the the Wellcome Sanger Institute to decode the genomes of all the eukaryotic species in the United Kingdom, thought to number about 66,000. Nature | 3 min read https://www.sciencemag.org/news/2018/11/poop-vault-human-feces-could-preserve-gut-biodiversity-and-help-treat- disease?utm_campaign=news_weekly_2018-11-02&et_rid=328058488&et_cid=2465523 Bacterial Heme-Based Sensors of Nitric Oxide Dominique E. -
The-Plant-Cell.Pdf
Levetin−McMahon: Plants II. Introduction to Plant 2. The Plant Cell © The McGraw−Hill and Society, Fifth Edition Life: Botanical Principles Companies, 2008 UNIT II CHAPTER OUTLINE Early Studies of Cells 20 The Cell Wall 22 The Protoplast 22 Membranes 22 Moving Into and Out of Cells 22 Organelles 23 A CLOSER LOOK 2.1 Origin of Chloroplasts and Mitochondria 25 The Nucleus 26 Cell Division 26 The Cell Cycle 26 Prophase 27 Metaphase 27 Anaphase 27 Telophase 27 Cytokinesis 27 Chapter Summary 30 Review Questions 30 Further Reading 30 KEY CONCEPTS 1. The Cell Theory establishes that the cell is the basic unit of life, that all living organisms are composed of cells, and that cells arise from preexisting cells. 2. Plant cells are eukaryotic, having an organized nucleus and membrane-bound organelles. 3. Substances can move into and out of cells by diffusion and osmosis. 4. Mitosis, followed by cytokinesis, results in two genetically identical daughter cells. Growth, replacement of cells, and asexual reproduction all depend on the process of cell division. CHAPTER 2 The Plant Cell Plantlets are produced by vegetative reproduction on the leaf margin of kalanchoe. Mitosis is the underlying cell division for vegetative or asexual reproduction. 19 Levetin−McMahon: Plants II. Introduction to Plant 2. The Plant Cell © The McGraw−Hill and Society, Fifth Edition Life: Botanical Principles Companies, 2008 20 UNIT II Introduction to Plant Life: Botanical Principles ll plants (and every other living organism) are com- posed of cells. In some algae and fungi, the whole A organism consists of a single cell, but angiosperms are complex multicellular organisms composed of many dif- ferent types of cells. -
Roles of Autophagy in Chloroplast Recycling☆
View metadata, citation and similar papers at core.ac.uk brought to you by CORE provided by Elsevier - Publisher Connector Biochimica et Biophysica Acta 1837 (2014) 512–521 Contents lists available at ScienceDirect Biochimica et Biophysica Acta journal homepage: www.elsevier.com/locate/bbabio Review Roles of autophagy in chloroplast recycling☆ Hiroyuki Ishida a,⁎, Masanori Izumi b,ShinyaWadaa, Amane Makino a,c a Graduate School of Agricultural Sciences, Tohoku University, Tsutsumidori-Amamiyamachi, Aoba-ku, Sendai 981-8555, Japan b Department of Environmental Life Sciences, Graduate School of Life Sciences, Tohoku University, Katahira, Aoba-ku, Sendai 980-8577, Japan c Core Research for Evolutional Science and Technology (CREST), Japan Science and Technology Agency, Chiyoda-ku, Tokyo 102-0076, Japan article info abstract Article history: Chloroplasts are the primary energy suppliers for plants, and much of the total leaf nitrogen is distributed to these Received 29 July 2013 organelles. During growth and reproduction, chloroplasts in turn represent a major source of nitrogen to be re- Received in revised form 1 November 2013 covered from senescing leaves and used in newly-forming and storage organs. Chloroplast proteins also can be Accepted 7 November 2013 an alternative substrate for respiration under suboptimal conditions. Autophagy is a process of bulk degradation Available online 19 November 2013 and nutrient sequestration that is conserved in all eukaryotes. Autophagy can selectively target chloroplasts as whole organelles and or as Rubisco-containing bodies that are enclosed by the envelope and specifically contain Keywords: Autophagy the stromal portion of the chloroplast. Although information is still limited, recent work indicates that chloroplast Chloroplast recycling via autophagy plays important roles not only in developmental processes but also in organelle quality Leaf senescence control and adaptation to changing environments. -
Role of Endodermal Cell Vacuoles in Shoot Gravitropism
J Plant Growth Regul 2002) 21:113±119 DOI: 10.1007/s003440010047 Role of Endodermal Cell Vacuoles in Shoot Gravitropism Takehide Kato,1 Miyo Terao Morita,2 and Masao Tasaka2,* 1Department of Botany, Graduate School of Science, Kyoto University, Sakyo-ku, Kyoto, 606-8502, Japan 2Graduate School of Biological Sciences, Nara Institute of Science and Technology, Nara, 630-0101, Japan ABSTRACT In higher plants, shoots and roots show negative endodermis would function as statoliths, just as in and positive gravitropism, respectively. Data from roots. surgical ablation experiments and analysis of starch The study of the sgr2 and zig/sgr4 mutants pro- de®cient mutants have led to the suggestion that vides new insights into the early steps of shoot columella cells in the root cap function as gravity gravitropism, which still remains unclear. SGR2 and perception cells. On the other hand, endodermal ZIG/SGR4 genes encode a phospholipase-like and a cells are believed to be the statocytes that is, gravity v-SNARE protein, respectively. Moreover, these perceiving cells) of shoots. Statocytes in shoots and genes are involved in vacuolar formation or func- roots commonly contain amyloplasts which sedi- tion. Thus, the vacuole must play an important role ment under gravity. Through genetic research with in amyloplast sedimentation because the sgr2 and Arabidopsis shoot gravitropism mutants, sgr1/scr and zig/sgr4 mutants display abnormal amyloplast sedi- sgr7/shr, it was determined that endodermal cells are mentation. essential for shoot gravitropism. Moreover, some starch biosynthesis genes and EAL1 are important for the formation and maturation of amyloplasts in Key words: Shoot gravitropism; Endodermis; shoot endodermis. -
Quantitative Analysis of the Grain Amyloplast Proteome Reveals
Ma et al. BMC Genomics (2018) 19:768 https://doi.org/10.1186/s12864-018-5174-z RESEARCH ARTICLE Open Access Quantitative analysis of the grain amyloplast proteome reveals differences in metabolism between two wheat cultivars at two stages of grain development Dongyun Ma1,2*† , Xin Huang1†, Junfeng Hou1, Ying Ma1, Qiaoxia Han1, Gege Hou1, Chenyang Wang1,2 and Tiancai Guo1 Abstract Background: Wheat (Triticum aestivum L.) is one of the world’s most important grain crops. The amyloplast, a specialized organelle, is the major site for starch synthesis and storage in wheat grain. Understanding the metabolism in amyloplast during grain development in wheat cultivars with different quality traits will provide useful information for potential yield and quality improvement. Results: Two wheat cultivars, ZM366 and YM49–198 that differ in kernel hardness and starch characteristics, were used to examine the metabolic changes in amyloplasts at 10 and 15 days after anthesis (DAA) using label-free-based proteome analysis. We identified 523 differentially expressed proteins (DEPs) between 10 DAA and 15 DAA, and 229 DEPs between ZM366 and YM49–198. These DEPs mainly participate in eight biochemical processes: carbohydrate metabolism, nitrogen metabolism, stress/defense, transport, energetics-related, signal transduction, protein synthesis/assembly/degradation, and nucleic acid-related processes. Among these proteins, the DEPs showing higher expression levels at 10 DAA are mainly involved in carbohydrate metabolism, stress/defense, and nucleic acid related processes, whereas DEPs with higher expression levels at 15 DAA are mainly carbohydrate metabolism, energetics-related, and transport-related proteins. Among the DEPs between the two cultivars, ZM366 had more up-regulated proteins than YM49–198, and these are mainly involved in carbohydrate metabolism, nucleic acid-related processes, and transport. -
ER Membrane-Localized Oxidoreductase Ero1 Is Required for Disulfide Bond Formation in the Rice Endosperm
ER membrane-localized oxidoreductase Ero1 is required for disulfide bond formation in the rice endosperm Yayoi Ondaa, Toshihiro Kumamarub, and Yasushi Kawagoea,1 aDivision of Plant Sciences, National Institute of Agrobiological Sciences, 2-1-2 Kannondai, Tsukuba, Ibaraki 305-8602, Japan; and bInstitute of Genetic Resources, Faculty of Agriculture, Kyushu University, Hakozaki, Fukuoka 812-8581, Japan Edited by Bob B. Buchanan, University of California, Berkeley, CA, and approved July 1, 2009 (received for review April 22, 2009) The developing endosperm of rice (Oryza sativa, Os) synthesizes a one constitutes the major small-molecule redox buffer in the ER. large amount of storage proteins on the rough (r)ER. The major The redox state of the ER is more oxidative than that of the cytosol storage proteins, glutelins and prolamins, contain either intra or in eukaryotic cells. The ratio of reduced glutathione to oxidized intermolecular disulfide bonds, and oxidative protein folding is nec- form ([glutathione (GSH)]/[oxidized glutathione (GSSG)]) ranges essary for the sorting of the proteins to the protein bodies. Here, we from 1:1 to 3:1 in the ER, whereas it ranges from 30:1 to 100:1 in investigated an electron transfer pathway for the formation of the cytosol (8). In the endosperm cells, which are devoted to the protein disulfide bonds in the rER of the rice endosperm, focusing on synthesis of disulfide-rich storage proteins, large amounts of reduc- the roles of the thiol-disulfide oxidoreductase, OsEro1. Confocal ing equivalents flux from the cytosol into the ER in the form of Cys microscopic analysis revealed that N-glycosylated OsEro1 is localized residues during the synthesis of storage proteins, and storage to the rER membrane in the subaleurone cells, and that targeting of proteins are sequestered from the ER lumen to the PBs. -
Bop8e Chapter03 REVISED W16 Part 1
Ray F. Evert • Susan E. Eichhorn Raven Biology of Plants Eighth Edition CHAPTER 3 The Plant Cell and the Cell Cycle © 2013 W. H. Freeman and Company Powerhouse of the Plant Cell • The chloroplast is the site where light energy is used to produce the organic molecules required by the plant cell • In this image, the flattened and stacked membranes of the grana can be seen inside the chloroplast • Chlorophyll and other pigments embedded in the chloroplast membranes capture the sun's energy, the first step in the process essential to life—photosynthesis Hooke's Microscope • The English microscopist Robert Hooke first used the term "cell" to refer to the small chambers he saw in magnified slices of cork • (a) One of Hooke's microscopes, made for him around 1670 • Light from an oil lamp (far left) was directed to the specimen through a water-filled glass globe that acted as a condenser • The specimen was mounted on a pin, just below the tip of the microscope • The microscope was focused by moving it up and down using a screw held to the stand by a clamp • (b) This drawing of two slices of cork appeared in Hooke's book, Micrographia, published in 1665 Chapter Overview • Prokaryotes and Eukaryotes • The Plant Cell: An Overview • Nucleus • Chloroplasts and Other Plastids • Mitochondria • Peroxisomes • Vacuoles • Endoplasmic Reticulum • Golgi Apparatus • Cytoskeleton • Flagella and Cilia • Cell Wall • The Cell Cycle • Interphase • Mitosis and Cytokinesis Main Points of this Chapter • The cell is the fundamental unit of life • Cells are of two fundamentally -
Imaging Amyloplasts in the Developing Endosperm of Barley and Rice
www.nature.com/scientificreports OPEN Imaging Amyloplasts in the Developing Endosperm of Barley and Rice Received: 28 September 2018 Ryo Matsushima & Hiroshi Hisano Accepted: 14 February 2019 Amyloplasts are plant-specifc organelles responsible for starch biosynthesis and storage. Inside Published: xx xx xxxx amyloplasts, starch forms insoluble particles, referred to as starch grains (SGs). SG morphology difers between species and SG morphology is particularly diverse in the endosperm of Poaceae plants, such as rice (Oryza sativa) and barley (Hordeum vulgare), which form compound SGs and simple SGs, respectively. SG morphology has been extensively imaged, but the comparative imaging of amyloplast morphology has been limited. In this study, SG-containing amyloplasts in the developing endosperm were visualized using stable transgenic barley and rice lines expressing amyloplast stroma-targeted green fuorescent protein fused to the transit peptide (TP) of granule-bound starch synthase I (TP- GFP). The TP-GFP barley and rice plants had elongated amyloplasts containing multiple SGs, with constrictions between the SGs. In barley, some amyloplasts were connected by narrow protrusions extending from their surfaces. Transgenic rice lines producing amyloplast membrane-localized SUBSTANDARD STARCH GRAIN6 (SSG6)-GFP were used to demonstrate that the developing amyloplasts contained multiple compound SGs. TP-GFP barley can be used to visualize the chloroplasts in leaves and other plastids in pollen and root in addition to the endosperm, therefore it provides as a useful tool to observe diverse plastids. Amyloplasts are a type of plastid surrounded by a double lipid bilayer of inner and outer envelope membranes1. Plants develop amyloplasts in storage organs such as the endosperm and tubers to biosynthesize and store glucose as starch.