Operator Guide, 144+ Pages, Pdf, Latex /Memoir Format
Total Page:16
File Type:pdf, Size:1020Kb
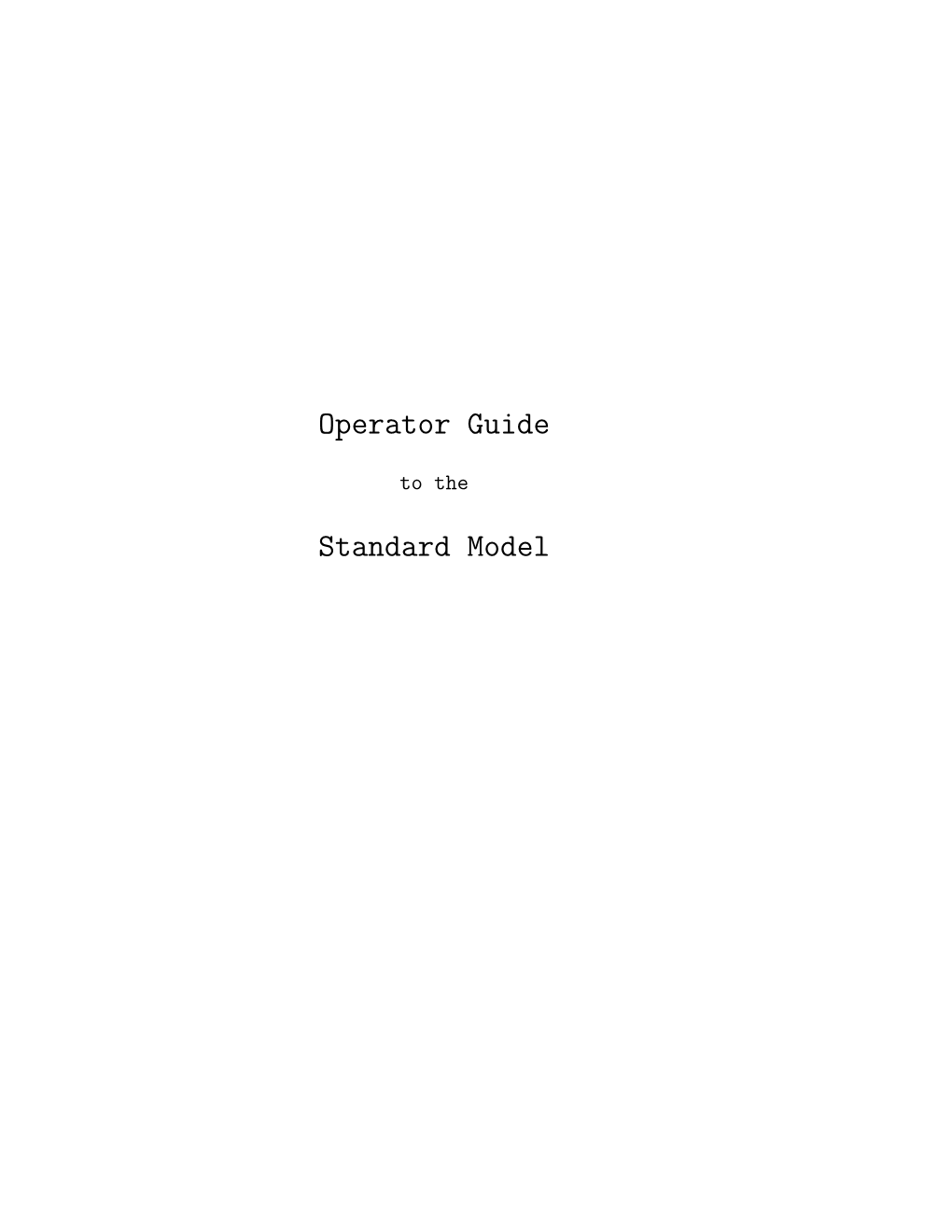
Load more
Recommended publications
-
Can We Rule out the Possibility of the Existence of an Additional Time Axis
Brian D. Koberlein Thomas J. Murray Time 2 ABSTRACT CAN WE RULE OUT THE POSSIBILITY THAT THERE IS MORE THAN ONE DIRECTION IN TIME? by Lynn Stephens The literature was examined to see if a proposal for the existence of additional time-like axes could be falsified. Some naïve large-time models are visualized and examined from the standpoint of simplicity and adequacy. Although the conclusion here is that the existence of additional time-like dimensions has not been ruled out, the idea is probably not falsifiable, as was first assumed. The possibility of multiple times has been dropped from active consideration by theorists less because of negative evidence than because it can lead to cumbersome models that introduce causality problems without providing any advantages over other models. Even though modern philosophers have made intriguing suggestions concerning the causality issue, usability and usefulness issues remain. Symmetry considerations from special relativity and from the conservation laws are used to identify restrictions on large-time models. In view of recent experimental findings, further research into Cramer’s transactional model is indicated. This document contains embedded graphic (.png and .jpg) and Apple Quick Time (.mov) files. The CD-ROM contains interactive Pacific Tech Graphing Calculator (.gcf) files. Time 3 NOTE TO THE READER The graphics in the PDF version of this document rely heavily on the use of color and will not print well in black and white. The CD-ROM attachment that comes with the paper version of this document includes: • The full-color PDF with QuickTime animation; • A PDF printable in black and white; • The original interactive Graphing Calculator files. -
Simulating Physics with Computers
International Journal of Theoretical Physics, VoL 21, Nos. 6/7, 1982 Simulating Physics with Computers Richard P. Feynman Department of Physics, California Institute of Technology, Pasadena, California 91107 Received May 7, 1981 1. INTRODUCTION On the program it says this is a keynote speech--and I don't know what a keynote speech is. I do not intend in any way to suggest what should be in this meeting as a keynote of the subjects or anything like that. I have my own things to say and to talk about and there's no implication that anybody needs to talk about the same thing or anything like it. So what I want to talk about is what Mike Dertouzos suggested that nobody would talk about. I want to talk about the problem of simulating physics with computers and I mean that in a specific way which I am going to explain. The reason for doing this is something that I learned about from Ed Fredkin, and my entire interest in the subject has been inspired by him. It has to do with learning something about the possibilities of computers, and also something about possibilities in physics. If we suppose that we know all the physical laws perfectly, of course we don't have to pay any attention to computers. It's interesting anyway to entertain oneself with the idea that we've got something to learn about physical laws; and if I take a relaxed view here (after all I'm here and not at home) I'll admit that we don't understand everything. -
Dirac Equation - Wikipedia
Dirac equation - Wikipedia https://en.wikipedia.org/wiki/Dirac_equation Dirac equation From Wikipedia, the free encyclopedia In particle physics, the Dirac equation is a relativistic wave equation derived by British physicist Paul Dirac in 1928. In its free form, or including electromagnetic interactions, it 1 describes all spin-2 massive particles such as electrons and quarks for which parity is a symmetry. It is consistent with both the principles of quantum mechanics and the theory of special relativity,[1] and was the first theory to account fully for special relativity in the context of quantum mechanics. It was validated by accounting for the fine details of the hydrogen spectrum in a completely rigorous way. The equation also implied the existence of a new form of matter, antimatter, previously unsuspected and unobserved and which was experimentally confirmed several years later. It also provided a theoretical justification for the introduction of several component wave functions in Pauli's phenomenological theory of spin; the wave functions in the Dirac theory are vectors of four complex numbers (known as bispinors), two of which resemble the Pauli wavefunction in the non-relativistic limit, in contrast to the Schrödinger equation which described wave functions of only one complex value. Moreover, in the limit of zero mass, the Dirac equation reduces to the Weyl equation. Although Dirac did not at first fully appreciate the importance of his results, the entailed explanation of spin as a consequence of the union of quantum mechanics and relativity—and the eventual discovery of the positron—represents one of the great triumphs of theoretical physics. -
Arxiv:Hep-Th/9603202V1 29 Mar 1996
SLAC–PUB–7115 March 1996 DISCRETE PHYSICS AND THE DIRAC EQUATION∗ Louis H. Kauffman Department of Mathematics, Statistics and Computer Science University of Illinois at Chicago 851 South Morgan Street, Chicago IL 60607-7045 and H. Pierre Noyes Stanford Linear Accelerator Center Stanford University, Stanford, CA 94309 Abstract We rewrite the 1+1 Dirac equation in light cone coordinates in two signif- icant forms, and solve them exactly using the classical calculus of finite differ- ences. The complex form yields “Feynman’s Checkerboard”—a weighted sum arXiv:hep-th/9603202v1 29 Mar 1996 over lattice paths. The rational, real form can also be interpreted in terms of bit-strings. Submitted to Physics Letters A. PACS: 03.65.Pm, 02.70.Bf Key words: discrete physics, choice sequences, Dirac equation, Feynman checker- board, calculus of finite differences, rational vs. complex quantum mechanics ∗Work partially supported by Department of Energy contract DE–AC03–76SF00515 and by the National Science Foundation under NSF Grant Number DMS-9295277. 1 Introduction In this paper we give explicit solutions to the Dirac equation for 1+1 space-time. These solutions are valid for discrete physics [1] using the calculus of finite differences, and they have as limiting values solutions to the Dirac equation using infinitesimal calculus. We find that the discrete solutions can be directly interpreted in terms of sums over lattice paths in discrete space-time. We document the relationship of this lattice-path with the checkerboard model of Richard Feynman [2]. Here we see how his model leads directly to an exact solution to the Dirac equation in discrete physics and thence to an exact continuum solution by taking a limit. -
Feynman Checkerboard an Intro to Algorithmic Quantum Field Theory
Feynman checkerboard An intro to algorithmic quantum field theory E. Akhmedova, M. Skopenkov, A. Ustinov, R. Valieva, A. Voropaev Summary. It turns out that rainbow patterns on soapbubbles and unbelievable laws of motion of electrons can be explained by means of one simple-minded model. It is a game, in which a checker moves on a checkerboard by certain simple rules, and we count the turnings. This ‘Feynman checkerboard’ can explain all phenomena in the world (with a serious proviso) except atomic nuclei and gravitation. We are going 0.007 to solve mathematical problems related to the game and discuss their 0.006 0.005 physical meaning; no knowledge of physics is assumed. 0.004 0.003 Main results. The main results are explicit formulae for 0.002 0.001 the percentage of light of given color reflected by a glass plate of ∙ Basic model from §1 given width (Problem 24); ∙ the probability to find an electron at a given point, if it wasemit- ted from the origin (Problem 15; see Figure 1). Although the results are stated in physical terms, they are mathemati- cal theorems, because we provide mathematical models of the physical phenomena in question, with all the objects defined rigorously. More precisely, a sequence of models with increasing precision. 0.00010 0.00008 Plan. We start with a basic model and upgrade it step by step 0.00006 0.00004 in each subsequent section. Before each upgrade, we summarize which 0.00002 physical question does it address, which simplifying assumptions does it resolve or impose additionally, and which experimental results does Model with mass from §3 it explain. -
Feynman Checkers: the Probability to Find an Electron Vanishes Nowhere Inside the Light Cone
Feynman checkers: the probability to find an electron vanishes nowhere inside the light cone Novikov Ivan Abstract We study Feynman checkers, the most elementary model of electron motion introduced by R. Feynman. For the model, we prove that the probability to find an electron vanishes nowhere inside the light cone. We also prove several results on the average electron velocity. In addition, we present a lot of identities related to the model. Keywords: Feynman checkerboard, quantum mechanics, average velocity, Dirac equation. 1 Introduction This paper is on the most elementary model of one-dimensional electron motion that is known as “Feynman checkers”. This model was introduced by R. Feynman around the 1950s and published in 1965; see [3, Problem 2.6]. Afterwards, a large amount of physical articles on the model appeared (see, for example, [1, 5, 6, 7, 9]). But the first mathematical work [10] on the subject appeared, apparently, only in 2020. We use the inessential modification of the model from [3] that was presented in [10]. Many properties of our model are parallel to usual quantum mechanics: there are analogues of Dirac equation (Proposition 1), probability/charge conservation (Proposition 4), Klein–Gordon equation [10, Proposition 7], Fourier integral [10, Propositions 12-13], concentration of measure on the light cone [10, Corollary 6] etc. Other striking properties have sharp contrast with both continuum quantum theory and the classical random walks: the (essentially) maximal electron velocity is strictly less than the speed of light [1, Theorem 1], [10, Theorem 1(B)]; adding absorbing boundary increases the probability of returning to the initial point [1, Theorems 8 and 10]. -
Inferences About Interactions: Fermions and the Dirac Equation
Inferences about Interactions: Fermions and the Dirac Equation Kevin H. Knuth Departments of Physics and Informatics University at Albany (SUNY) Albany NY 12222, USA November 10, 2018 Abstract At a fundamental level every measurement process relies on an interac- tion where one entity influences another. The boundary of an interaction is given by a pair of events, which can be ordered by virtue of the interac- tion. This results in a partially ordered set (poset) of events often referred to as a causal set. In this framework, an observer can be represented by a chain of events. Quantification of events and pairs of events, referred to as intervals, can be performed by projecting them onto an observer chain, or even a pair of observer chains, which in specific situations leads to a Minkowski metric replete with Lorentz transformations. We illustrate how this framework of interaction events gives rise to some of the well- known properties of the Fermions, such as Zitterbewegung. We then take this further by making inferences about events, which is performed by employing the process calculus, which coincides with the Feynman path integral formulation of quantum mechanics. We show that in the 1+1 dimensional case this results in the Feynman checkerboard model of the Dirac equation describing a Fermion at rest. 1 Introduction This paper summarizes the synthesis of three distinct research threads which arXiv:1212.2332v1 [quant-ph] 11 Dec 2012 were inspired by the quantification of Boolean algebra by Cox [1]. The first represents the current perspective of the quantification of the Boolean lattice of logical statements, which results in probability theory [2, 3]. -
Downloaded the Top 100 the Seed to This End
PROC. OF THE 11th PYTHON IN SCIENCE CONF. (SCIPY 2012) 11 A Tale of Four Libraries Alejandro Weinstein‡∗, Michael Wakin‡ F Abstract—This work describes the use some scientific Python tools to solve One of the contributions of our research is the idea of rep- information gathering problems using Reinforcement Learning. In particular, resenting the items in the datasets as vectors belonging to a we focus on the problem of designing an agent able to learn how to gather linear space. To this end, we build a Latent Semantic Analysis information in linked datasets. We use four different libraries—RL-Glue, Gensim, (LSA) [Dee90] model to project documents onto a vector space. NetworkX, and scikit-learn—during different stages of our research. We show This allows us, in addition to being able to compute similarities that, by using NumPy arrays as the default vector/matrix format, it is possible to between documents, to leverage a variety of RL techniques that integrate these libraries with minimal effort. require a vector representation. We use the Gensim library to build Index Terms—reinforcement learning, latent semantic analysis, machine learn- the LSA model. This library provides all the machinery to build, ing among other options, the LSA model. One place where Gensim shines is in its capability to handle big data sets, like the entirety of Wikipedia, that do not fit in memory. We also combine the vector Introduction representation of the items as a property of the NetworkX nodes. In addition to bringing efficient array computing and standard Finally, we also use the manifold learning capabilities of mathematical tools to Python, the NumPy/SciPy libraries provide sckit-learn, like the ISOMAP algorithm [Ten00], to perform some an ecosystem where multiple libraries can coexist and interact. -
Path-Integral Solution of the One-Dimensional Dirac Quantum Cellular Automaton
Physics Letters A 378 (2014) 3165–3168 Contents lists available at ScienceDirect Physics Letters A www.elsevier.com/locate/pla Path-integral solution of the one-dimensional Dirac quantum cellular automaton a,b a a,b a, Giacomo Mauro D’Ariano , Nicola Mosco , Paolo Perinotti , Alessandro Tosini ∗ a QUIT group, Dipartimento di Fisica, via Bassi 6, Pavia, 27100, Italy b INFN Gruppo IV, Sezione di Pavia, via Bassi 6, Pavia, 27100, Italy a r t i c l e i n f o a b s t r a c t Article history: Quantum cellular automata, which describe the discrete and exactly causal unitary evolution of a lattice Received 4 June 2014 of quantum systems, have been recently considered as a fundamental approach to quantum field theory Received in revised form 23 August 2014 and a linear automaton for the Dirac equation in one dimension has been derived. In the linear case a Accepted 12 September 2014 quantum cellular automaton is isomorphic to a quantum walk and its evolution is conveniently formu- Available online 16 September 2014 lated in terms of transition matrices. The semigroup structure of the matrices leads to a new kind of Communicated by P.R. Holland discrete path-integral, different from the well known Feynman checkerboard one, that is solved analyti- Keywords: cally in terms of Jacobi polynomials of the arbitrary mass parameter. Quantum cellular automata © 2014 Elsevier B.V. All rights reserved. Quantum walks Dirac quantum cellular automaton Discrete path-integral 1. Introduction replacing the quantum state with a quantum field on the lattice, a QW describes a QCA that is linear in the field (providing the dis- The simplest example of discrete evolution of physical systems crete evolution of non-interacting particles with a given statistics). -
From Sets to Quarks: Deriving the Standard Model Plus Gravitation from Simple Operations on Finite Sets Frank D
View metadata, citation and similar papers at core.ac.uk brought to you by CORE provided by CERN Document Server hep-ph/9708379 TS-TH-97-1 August 1997, Revised June 1998 From Sets to Quarks: Deriving the Standard Model plus Gravitation from Simple Operations on Finite Sets Frank D. (Tony) Smith, Jr. e-mail: [email protected] and [email protected] P. O. Box 370, Cartersville, GA 30120 USA WWW URLs http://galaxy.cau.edu/tsmith/TShome.html and http://www.innerx.net/personal/tsmith/TShome.html Abstract From sets and simple operations on sets, a Feynman Checkerboard physics model is constructed that allows computation of force strength constants and constituent mass ratios of elementary particles, with a Lagrangian structure that gives a Higgs scalar particle mass of about 146 GeV and a Higgs scalar field vacuum expectation value of about 252 GeV, giving a tree level constituent Truth Quark (top quark) mass of roughly 130 GeV, which is (in my opinion) supported by dileptonic events and some semileptonic events. See http://galaxy.cau.edu/tsmith/HDFCmodel.html and http://www.innerx.net/personal/tsmith/HDFCmodel.html Chapter 1 - Introduction. Chapter 2 - From Sets to Clifford Algebras. Chapter 3 - Octonions and E8 lattices. Chapter 4 - E8 spacetime and particles. Chapter 5 - HyperDiamond Lattices. Chapter 6 - Internal Symmetry Space. Chapter 7 - Feynman Checkerboards. Chapter 8 - Charge = Amplitude to Emit Gauge Boson. Chapter 9 - Mass = Amplitude to Change Direction. Chapter 10 - Protons, Pions, and Physical Gravitons. Contents 1 Introduction. 1 1.1ParticleMassesandForceConstants............... 2 1.1.1 Confined Quark Hadrons. .............. 4 1.2D4-D5-E6Lagrangianand146GeVHiggs........... -
Feynman Checkerboard an Elementary Mathematical Model in Quantum Theory
Feynman checkerboard An elementary mathematical model in quantum theory E. Akhmedova, M. Skopenkov, A. Ustinov, R. Valieva, A. Voropaev Summary. The course is devoted to the most elementary model of electron motion suggested by R.Feynman. It is a game, in which a checker moves on a checkerboard by certain simple rules, and we count the turnings. It turns out that this simple combinatorial model demonstrates visually many basic ideas of quantum theory. We are going to solve mathematical problems related to the game and discuss 0.014 their physical meaning; no knowledge of physics is assumed. 0.012 0.010 0.008 Main results. The main results are explicit formulae for 0.006 0.004 the percentage of light of given color reflected by a glass plate of 0.002 given width (Problem 26); Basic model from §1 the probability to find an electron in a given square, if it was emitted from the origin and moves in a fixed plane (Problem 15; see Figure 1). Although the results are stated in physical terms, they are mathemati- 0.0009 cal theorems, because we provide mathematical models of the physical 0.0008 0.0007 phenomena in question, with all the objects defined rigorously. More 0.0006 0.0005 precisely, a sequence of models with increasing precision. 0.0004 0.0003 Plan. We start with a basic model and upgrade it step by step 0.0002 in each subsequent section. Before each upgrade, we summarize which 0.0001 physical question does it address, which simplifying assumptions does Model with mass from §3 it resolve or impose additionally, and which experimental results does it explain. -
Quantum Lattice Boltzmann Is a Quantum Walk Sauro Succi1*, François Fillion-Gourdeau2 and Silvia Palpacelli3
Succi et al. EPJ Quantum Technology (2015)2:12 DOI 10.1140/epjqt/s40507-015-0025-1 R E S E A R C H Open Access Quantum lattice Boltzmann is a quantum walk Sauro Succi1*, François Fillion-Gourdeau2 and Silvia Palpacelli3 *Correspondence: [email protected] Abstract 1IAC-CNR, Istituto per le Applicazioni del Calcolo ‘Mauro Numerical methods for the 1-D Dirac equation based on operator splitting and on the Picone’, Via dei Taurini, 19, Rome, quantum lattice Boltzmann (QLB) schemes are reviewed. It is shown that these 00185, Italy discretizations fall within the class of quantum walks, i.e. discrete maps for complex Full list of author information is available at the end of the article fields, whose continuum limit delivers Dirac-like relativistic quantum wave equations. The correspondence between the quantum walk dynamics and these numerical schemes is given explicitly, allowing a connection between quantum computations, numerical analysis and lattice Boltzmann methods. The QLB method is then extended to the Dirac equation in curved spaces and it is demonstrated that the quantum walk structure is preserved. Finally, it is argued that the existence of this link between the discretized Dirac equation and quantum walks may be employed to simulate relativistic quantum dynamics on quantum computers. Keywords: quantum walks; Dirac equation; lattice Boltzmann; operator splitting 1 Introduction A quantum walk (QW) is defined as the quantum analogue of a classical random walk, where the ‘quantum walker’ is in a superposition of states instead of being described by a probability distribution. One of the earliest realization of this concept was proposed by Feynman as a discrete version of the massive Dirac equation [].