Structural and Functional Analysis of Immunity
Total Page:16
File Type:pdf, Size:1020Kb
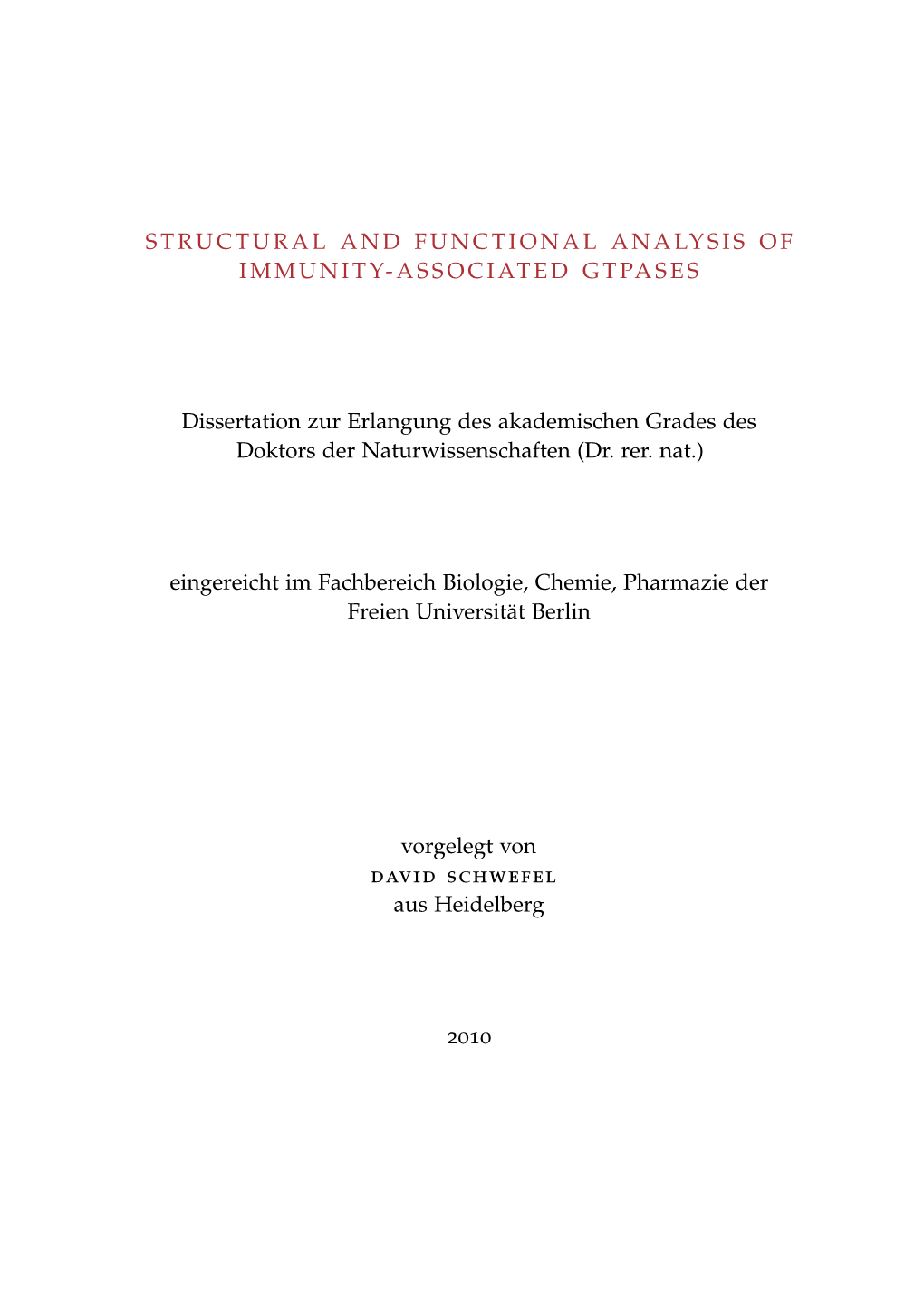
Load more
Recommended publications
-
IA-2 Autoantibodies in Incident Type I Diabetes Patients Are Associated with a Polyadenylation Signal Polymorphism in GIMAP5
Genes and Immunity (2007) 8, 503–512 & 2007 Nature Publishing Group All rights reserved 1466-4879/07 $30.00 www.nature.com/gene ORIGINAL ARTICLE IA-2 autoantibodies in incident type I diabetes patients are associated with a polyadenylation signal polymorphism in GIMAP5 J-H Shin1, M Janer2, B McNeney1, S Blay1, K Deutsch2, CB Sanjeevi3, I Kockum4,A˚ Lernmark5 and J Graham1, on behalf of the Swedish Childhood Diabetes and the Diabetes Incidence in Sweden Study Groups 1Department of Statistics and Actuarial Science, Simon Fraser University, Burnaby, British Columbia, Canada; 2Institute for Systems Biology, Seattle, WA, USA; 3Department of Molecular Medicine and Surgery, Karolinska Institutet, Stockholm, Sweden; 4Department of Clinical Neurosciences, Karolinska Institutet, Stockholm, Sweden and 5Department of Medicine, Robert H. Williams Laboratory, University of Washington, Seattle, WA, USA In a large case-control study of Swedish incident type I diabetes patients and controls, 0–34 years of age, we tested the hypothesis that the GIMAP5 gene, a key genetic factor for lymphopenia in spontaneous BioBreeding rat diabetes, is associated with type I diabetes; with islet autoantibodies in incident type I diabetes patients or with age at clinical onset in incident type I diabetes patients. Initial scans of allelic association were followed by more detailed logistic regression modeling that adjusted for known type I diabetes risk factors and potential confounding variables. The single nucleotide polymorphism (SNP) rs6598, located in a polyadenylation signal of GIMAP5, was associated with the presence of significant levels of IA-2 autoantibodies in the type I diabetes patients. Patients with the minor allele A of rs6598 had an increased prevalence of IA-2 autoantibody levels compared to patients without the minor allele (OR ¼ 2.2; Bonferroni-corrected P ¼ 0.003), after adjusting for age at clinical onset (P ¼ 8.0 Â 10À13) and the numbers of HLA-DQ A1*0501-B1*0201 haplotypes (P ¼ 2.4 Â 10 À5) and DQ A1*0301-B1*0302 haplotypes (P ¼ 0.002). -
An Investigation Into the Genetic Architecture of Multiple System Atrophy and Familial Parkinson's Disease
An investigation into the genetic architecture of multiple system atrophy and familial Parkinson’s disease By Monica Federoff A thesis submitted to University College London for the degree of Doctor of Philosophy Laboratory of Neurogenetics, Department of Molecular Neuroscience, Institute of Neurology, University College London (UCL) 2 I, Monica Federoff, confirm that the work presented in this thesis is my own. Information derived from other sources and collaborative work have been indicated appropriately. Signature: Date: 09/06/2016 3 Acknowledgements: When I first joined the Laboratory of Neurogenetics (LNG), NIA, NIH as a summer intern in 2008, I had minimal experience working in a laboratory and was both excited and anxious at the prospect of it. From my very first day, Dr. Andrew Singleton was incredibly welcoming and introduced me to my first mentor, Dr. Javier Simon- Sanchez. Within just ten weeks working in the lab, both Dr. Singleton and Dr. Simon- Sanchez taught me the fundamental skills in an encouraging and supportive environment. I quickly got to know others in the lab, some of whom are still here today, and I sincerely appreciate their help with my assimilation into the LNG. After returning for an additional summer and one year as an IRTA postbac, I was honored to pursue a PhD in such an intellectually stimulating and comfortable environment. I am so grateful that Dr. Singleton has been such a wonderful mentor, as he is not only a brilliant scientist, but also extremely personable and approachable. If I inquire about meeting with him, he always manages to make time in his busy schedule and provides excellent guidance and mentorship. -
Identification of Potential Key Genes and Pathway Linked with Sporadic Creutzfeldt-Jakob Disease Based on Integrated Bioinformatics Analyses
medRxiv preprint doi: https://doi.org/10.1101/2020.12.21.20248688; this version posted December 24, 2020. The copyright holder for this preprint (which was not certified by peer review) is the author/funder, who has granted medRxiv a license to display the preprint in perpetuity. All rights reserved. No reuse allowed without permission. Identification of potential key genes and pathway linked with sporadic Creutzfeldt-Jakob disease based on integrated bioinformatics analyses Basavaraj Vastrad1, Chanabasayya Vastrad*2 , Iranna Kotturshetti 1. Department of Biochemistry, Basaveshwar College of Pharmacy, Gadag, Karnataka 582103, India. 2. Biostatistics and Bioinformatics, Chanabasava Nilaya, Bharthinagar, Dharwad 580001, Karanataka, India. 3. Department of Ayurveda, Rajiv Gandhi Education Society`s Ayurvedic Medical College, Ron, Karnataka 562209, India. * Chanabasayya Vastrad [email protected] Ph: +919480073398 Chanabasava Nilaya, Bharthinagar, Dharwad 580001 , Karanataka, India NOTE: This preprint reports new research that has not been certified by peer review and should not be used to guide clinical practice. medRxiv preprint doi: https://doi.org/10.1101/2020.12.21.20248688; this version posted December 24, 2020. The copyright holder for this preprint (which was not certified by peer review) is the author/funder, who has granted medRxiv a license to display the preprint in perpetuity. All rights reserved. No reuse allowed without permission. Abstract Sporadic Creutzfeldt-Jakob disease (sCJD) is neurodegenerative disease also called prion disease linked with poor prognosis. The aim of the current study was to illuminate the underlying molecular mechanisms of sCJD. The mRNA microarray dataset GSE124571 was downloaded from the Gene Expression Omnibus database. Differentially expressed genes (DEGs) were screened. -
TAL1-Mediated Epigenetic Modifications Down-Regulate the Expression of GIMAP Family in Non-Small Cell Lung Cancer
bioRxiv preprint doi: https://doi.org/10.1101/739870; this version posted August 20, 2019. The copyright holder for this preprint (which was not certified by peer review) is the author/funder, who has granted bioRxiv a license to display the preprint in perpetuity. It is made available under aCC-BY-NC-ND 4.0 International license. TAL1-mediated epigenetic modifications down-regulate the expression of GIMAP family in non-small cell lung cancer Zhongxiang Tang, Lili Wang, Pei Dai, Pinglang Ruan, Dan Liu, Yurong Tan* Department of Microbiology, Xiangya School of Medicine, Central South University, Changsha 410078, Hunan, China. *Corresponding author: Yurong Tan. Department of Microbiology, Xiangya School of Medicine, Central South University, Changsha 410078, Hunan, China. Tel: 86-73182355003; Fax: 86-73182355003; E-mail: [email protected]. bioRxiv preprint doi: https://doi.org/10.1101/739870; this version posted August 20, 2019. The copyright holder for this preprint (which was not certified by peer review) is the author/funder, who has granted bioRxiv a license to display the preprint in perpetuity. It is made available under aCC-BY-NC-ND 4.0 International license. Abstract Backgroud: The study was designed to explore the role of GIMAP family in non-small cell lung cancer (NSCLC) and its possible expression regulation mechanisms using existing biological databases including encyclopedia of DNA elements (ENCODE), gene expression omnibus (GEO), and the cancer genome atlas (TCGA). Methods: Lung squamous cell carcinoma (LUSC) and lung adenocarcinoma (LUAD) were used to evaluate the expression of GIMAPs in TCGA. Five NSCLC datasets were then selected from GEO for validation. -
A Critical Role for Gimap5 in CD4+ T Cell Homeostasis and Maintenance of Peripheral Immune Tolerance
A Critical Role for Gimap5 in CD4+ T cell Homeostasis and Maintenance of Peripheral Immune Tolerance A dissertation submitted to the Graduate School of the University of Cincinnati in partial fulfillment of the requirements for the degree of Doctor of Philosophy (Ph.D.) in the Immunobiology Graduate Program of the College of Medicine 2013 by Halil Ibrahim Aksoylar B.S., Middle East Technical University, Turkey, 2003 M.S., Sabanci University, Turkey, 2005 Committee Chair: Kasper Hoebe, Ph.D. Christopher Karp, M.D Edith Janssen, Ph.D. Julio Aliberti, Ph.D. David Plas, Ph.D. Abstract T cell lymphopenia is a condition which arises from defects in T cell development and/or peripheral homeostatic mechanisms. Importantly, lymphopenia is often associated with T cell-mediated pathology in animal models and in patients with autoimmune disease. In this thesis, using an ENU mutagenesis approach, we identified sphinx mice which presented severe lymphopenia due to a missense mutation in Gimap5. Characterization of Gimap5sph/sph mice revealed that Gimap5 is necessary for the development of NK and CD8+ T cells, and is required for the maintenance of peripheral CD4+ T and B cell populations. Moreover, Gimap5-deficient mice developed spontaneous colitis which resulted in early mortality. Gimap5sph/sph CD4+ T cells presented progressive lymphopenia-induced proliferation (LIP), became Th1/Th17 polarized, and mediated the development of colitis. Furthermore, Gimap5sph/sph FoxP3+ regulatory T cells became selectively reduced in the mesenteric lymph nodes and adoptive transfer of wild type regulatory T cells prevented colitis in Gimap5-deficient mice. Importantly, the expression of Foxo transcription factors, which play a critical role in T quiescence and Treg function, was progressively lost in the absence of Gimap5 suggesting a link between Gimap5 deficiency and loss of immunological tolerance. -
Ldheatmap (Version 0.9-1): Example of Adding Tracks
LDheatmap (Version 0.9-1): Example of Adding Tracks Jinko Graham and Brad McNeney December 5, 2012 1 Introduction As of version 0.9, LDheatmap allows users to “flip” the heatmap below a horizon- tal line in the style of Haploview. This extension was made so that “tracks” of genomic annotation information could be placed above the genetic map. Facili- ties for adding tracks are illustrated in this vignette. Limitations of the current implementation are discussed briefly in Section 6.2 2 Getting Started First load the package, the example dataset CIMAP5.CEU, and an R data file that contains two LDheatmap objects and one picture that will be used in the vignette. > library(LDheatmap) > data(GIMAP5.CEU) > load(system.file("extdata/addTracks.RData",package="LDheatmap")) The object GIMAP5.CEU is is a list with two elements: snp.data and snp.support. snp.data is a snp.matrix object from the chopsticks package, containing the SNP genotypes. Rows correspond to subjects and columns correspond to SNPs. snp.support is a data frame whose rows correspond to SNPs and whose columns give information on the SNPs, such as their alleles and genomic location. The help file help("GIMAP5.CEU") gives full details. In addition to GIMAP5.CEU, you should have the LDheatmap objects llGenes and llGenesRecomb in your workspace. These objects are the heatmap with tracks for genes and recombi- nation rates added incrementally. Adding these tracks requires fetching infor- mation from the UCSC genome browser, which requires an internet connection and can be time-consuming. These objects are provided so that users can see the track-annotated heatmaps without having to wait. -
The GIMAP Family Proteins: an Incomplete Puzzle
REVIEW published: 31 May 2021 doi: 10.3389/fimmu.2021.679739 The GIMAP Family Proteins: An Incomplete Puzzle Marc-Andre´ Limoges, Maryse Cloutier, Madhuparna Nandi, Subburaj Ilangumaran and Sheela Ramanathan* Department of Immunology and Cell Biology, Faculty of Medicine and Health Sciences, Universite´ de Sherbrooke and CRCHUS, Sherbrooke, QC, Canada Overview: Long-term survival of T lymphocytes in quiescent state is essential to maintain their cell numbers in secondary lymphoid organs and in peripheral circulation. In the BioBreeding diabetes-prone strain of rats (BB-DP), loss of functional GIMAP5 (GTPase of the immune associated nucleotide binding protein 5) results in profound peripheral T lymphopenia. This discovery heralded the identification of a new family of proteins initially called Immune-associated nucleotide binding protein (IAN) family. In this review we will use Edited by: ‘GIMAP’ to refer to this family of proteins. Recent studies suggest that GIMAP proteins Thomas Herrmann, may interact with each other and also be involved in the movement of the cellular cargo Julius Maximilian University of Würzburg, Germany along the cytoskeletal network. Here we will summarize the current knowledge on the Reviewed by: characteristics and functions of GIMAP family of proteins. Oliver Daumke, Max Delbrueck Center for Molecular Keywords: GIMAP5, gimap, lymphopenia, AIG domain, T lymphocyte, B cells Medicine, Germany Tiina Henttinen, University of Turku, Finland INTRODUCTION *Correspondence: In the BioBreeding diabetes-prone strain of rats (BB-DP), the recessive lyp mutation causes a Sheela Ramanathan profound loss of T lymphocytes in secondary lymphoid organs (1). Positional cloning of the gene [email protected] responsible for the lymphopenic phenotype in the BB-DP rats independently by two groups led to Specialty section: the discovery of a family of proteins that are conserved in vertebrates (2, 3). -
GIMAP5 (Gtpase of Immune Associated Nucleotide Binding Protein 5)
UNIVERSITÉ DE SHERBROOKE Régulation de l’apoptose des lymphocytes T par GIMAP5 (GTPase of Immune Associated Nucleotide Binding Protein 5) Par Xi-Lin Chen Département de pédiatrie, programme d’immunologie Thèse présentée à la Faculté de médecine et des sciences de la santé en vue de l’obtention du grade de philosophiae doctor (Ph.D) en Immunologie Sherbrooke, Québec, CANADA Le 01 Mai 2015 Membres du jury d’évaluation Dre Sheela Ramanathan, directrice, Département de pédiatrie, Service d’immunologie- Allergologie Dre Jana Stankova, co-directrice, Département de pédiatrie, Service d’immunologie-Allergologie Dr Subburaj Ilangumaran, Département de pédiatrie, Service d’immunologie-Allergologie Dr Guylain Boulay, Département de pharmacologie Dr Woong-Kyung Suh, Programme de biologie moléculaire, Université de Montréal © Xi-Lin Chen, 2015 Résumé Régulation de l’apoptose des lymphocytes T par GIMAP5 (GTPase of Immune Associated Nucleotide Binding Protein 5) Par Xi-Lin Chen Département de pédiatrie, programme d’immunologie, Thèse présentée à la Faculté de médecine et des sciences de la santé en vue de l’obtention du diplôme de doctorante (Ph.D.) en immunologie Faculté de médecine et des sciences de la santé, Université de Sherbrooke, Sherbrooke, Québec, Canada, J1H 5N4 La survie à long terme des lymphocytes T en état de repos est essentielle pour maintenir leurs nombres dans les organes lymphoïdes secondaires. Le récepteur antigénique des cellules T (TCR) en contact avec les peptides du soi / CMH et en synergie avec l'IL-7 induit des signaux anti-apoptotiques pour favoriser la survie des cellules T. Ces stimuli extrinsèques sont également impliqués dans le métabolisme et la survie des cellules T grâce à la régulation de plusieurs voies de signalisation dont la voie phosphatidyl-inositol-3 kinase (PI3K) /AKT. -
Human Immunodeficiency Reveals GIMAP5 As Lymphocyte-Specific Regulator of Senescence
bioRxiv preprint doi: https://doi.org/10.1101/2021.02.22.432146; this version posted February 23, 2021. The copyright holder for this preprint (which was not certified by peer review) is the author/funder. All rights reserved. No reuse allowed without permission. Human immunodeficiency reveals GIMAP5 as lymphocyte-specific regulator of senescence Ann Y. Park1†, Michael Leney-Greene1,2†, Matthew Lynberg1, Xijin Xu1, Lixin Zheng1, Yu Zhang2, Helen Matthews1, Brittany Chao1, Aaron Morawski1, Ping Jiang1, Jahnavi Aluri1, EliF Karakoc Aydiner5, Ayca Kiykim5, John Pascall7, Isil Barlan8, Sinan Sari9, Geoff Butcher7, V. Koneti Rao10, Richard Lifton6, SaFa Baris5, Ahmet Ozen5, Silvia Vilarinho3,4, Helen Su2, Michael J. Lenardo1* Affiliations: 1 Laboratory of Immune System Biology and Clinical Genomics Program, National Institute of Allergy and InFectious Diseases, National Institutes oF Health, Bethesda, MD 20892, USA 2 Laboratory of Host Defenses, National Institute of Allergy and Infectious Diseases, National Institutes of Health, Bethesda, MD 20892, USA 3 Section oF Digestive Diseases, Department oF Internal Medicine, Yale School oF Medicine, New Haven, CT 06519, USA 4 Departments oF Pathology, Yale School oF Medicine, New Haven, CT 06519, USA 5 Division oF Pediatric Allergy and Immunology, Marmara University, Fevzi Çakmak Mah. No: 41, Pendik, Istanbul, Turkey 6 Department oF Genetics, Yale School oF Medicine, Yale University, New Haven, CT 06520, USA 7 Laboratory of Lymphocyte Signaling and Development, The Babraham Institute, Cambridge, United Kingdom 8 The Isil Berat Barlan Center For Translational Medicine, Division oF Pediatric Allergy and Immunology, Marmara University, Fevzi Çakmak Mah. No: 41, Pendik, Istanbul, Turkey 9 Department oF Pediatric Gastroenterology, Gazi University Faculty oF Medicine, Ankara, Turkey 10 Laboratory of Clinical Immunology & Microbiology, National Institute oF Allergy and InFectious Diseases, National Institutes oF Health, Bethesda, MD 20892, USA * Correspondence to: [email protected] † These authors contributed equally: Ann Y. -
Apoptosis Regulator Gimap4/IAN1 a Natural Hypomorphic Variant Of
A Natural Hypomorphic Variant of the Apoptosis Regulator Gimap4/IAN1 Christine Carter, Carine Dion, Silke Schnell, W. John Coadwell, Margaret Graham, Lucy Hepburn, Geoffrey This information is current as Morgan, Amanda Hutchings, John C. Pascall, Heinz Jacobs, of September 26, 2021. J. Ross Miller and Geoffrey W. Butcher J Immunol 2007; 179:1784-1795; ; doi: 10.4049/jimmunol.179.3.1784 http://www.jimmunol.org/content/179/3/1784 Downloaded from References This article cites 36 articles, 18 of which you can access for free at: http://www.jimmunol.org/content/179/3/1784.full#ref-list-1 http://www.jimmunol.org/ Why The JI? Submit online. • Rapid Reviews! 30 days* from submission to initial decision • No Triage! Every submission reviewed by practicing scientists • Fast Publication! 4 weeks from acceptance to publication by guest on September 26, 2021 *average Subscription Information about subscribing to The Journal of Immunology is online at: http://jimmunol.org/subscription Permissions Submit copyright permission requests at: http://www.aai.org/About/Publications/JI/copyright.html Email Alerts Receive free email-alerts when new articles cite this article. Sign up at: http://jimmunol.org/alerts The Journal of Immunology is published twice each month by The American Association of Immunologists, Inc., 1451 Rockville Pike, Suite 650, Rockville, MD 20852 Copyright © 2007 by The American Association of Immunologists All rights reserved. Print ISSN: 0022-1767 Online ISSN: 1550-6606. The Journal of Immunology A Natural Hypomorphic Variant of the Apoptosis Regulator Gimap4/IAN11 Christine Carter,* Carine Dion,2* Silke Schnell,2† W. John Coadwell,* Margaret Graham,* Lucy Hepburn,* Geoffrey Morgan,* Amanda Hutchings,* John C. -
Serrano Daniel Phd 2017.Pdf
1 Université de Sherbrooke GIMAP5 influence la survie des cellules T naïves en participant à la régulation du calcium emmagasiné dans les organites Par Daniel Serrano Programme d’immunologie Thèse présentée à la Faculté de médecine et des sciences de la santé en vue de l’obtention du grade de Philosophiae Doctor (Ph.D.) en immunologie Sherbrooke, Québec, Canada Mars 2017 Membres du jury d’évaluation Sheela Ramanathan, Programme d’immunologie, Université de Sherbrooke Christine Lavoie, Département de pharmacologie, Université de Sherbrooke Patrick McDonald, Programme d’immunologie, Université de Sherbrooke Mercedes Rincon, Department of Immunobiology, University of Vermont © Daniel Serrano, 2017 i Live so that when your children think of fairness, caring, and integrity, they think of you. H. Jackson Brown, Jr. ii RÉSUMÉ GIMAP5 influence la survie des cellules T naïves en participant à la régulation du calcium emmagasiné dans les organites Par Daniel Serrano Programme d’immunologie Thèse présentée à la Faculté de médecine et des sciences de la santé en vue de l’obtention du diplôme de Philosophiae Doctor (Ph.D.) en Immunologie, Faculté de médecine et des sciences de la santé, Université de Sherbrooke, Sherbrooke, Québec, Canada, J1H 5N4 La survie des cellules T naïves est essentielle au bon fonctionnement du système immunitaire à long terme. Les rats BBDP (Bio-breeding Diabetes prone) sont caractérisés par une haute prédisposition au développement du diabète ainsi que par une diminution significative du nombre de cellules T naïves. Ces rats comportent une mutation de type décalage de lecture dans le gène codant pour «GTPase Immunity-Associated Protein 5» (Gimap5) ce qui entraine l’apoptose des lymphocytes T. -
Attractor Metafeatures and Their Application in Biomolecular Data Analysis
Attractor Metafeatures and Their Application in Biomolecular Data Analysis Tai-Hsien Ou Yang Submitted in partial fulfillment of the requirements for the degree of Doctor of Philosophy in the Graduate School of Arts and Sciences COLUMBIA UNIVERSITY 2018 ©2018 Tai-Hsien Ou Yang All rights reserved ABSTRACT Attractor Metafeatures and Their Application in Biomolecular Data Analysis Tai-Hsien Ou Yang This dissertation proposes a family of algorithms for deriving signatures of mutually associated features, to which we refer as attractor metafeatures, or simply attractors. Specifically, we present multi-cancer attractor derivation algorithms, identifying correlated features in signatures from multiple biological data sets in one analysis, as well as the groups of samples or cells that exclusively express these signatures. Our results demonstrate that these signatures can be used, in proper combinations, as biomarkers that predict a patient’s survival rate, based on the transcriptome of the tumor sample. They can also be used as features to analyze the composition of the tumor. Through analyzing large data sets of 18 cancer types and three high-throughput platforms from The Cancer Genome Atlas (TCGA) PanCanAtlas Project and multiple single-cell RNA-seq data sets, we identified novel cancer attractor signatures and elucidated the identity of the cells that express these signatures. Using these signatures, we developed a prognostic biomarker for breast cancer called the Breast Cancer Attractor Metagenes (BCAM) biomarker as well as a software platform