L-Carnitine in Drosophila: a Review
Total Page:16
File Type:pdf, Size:1020Kb
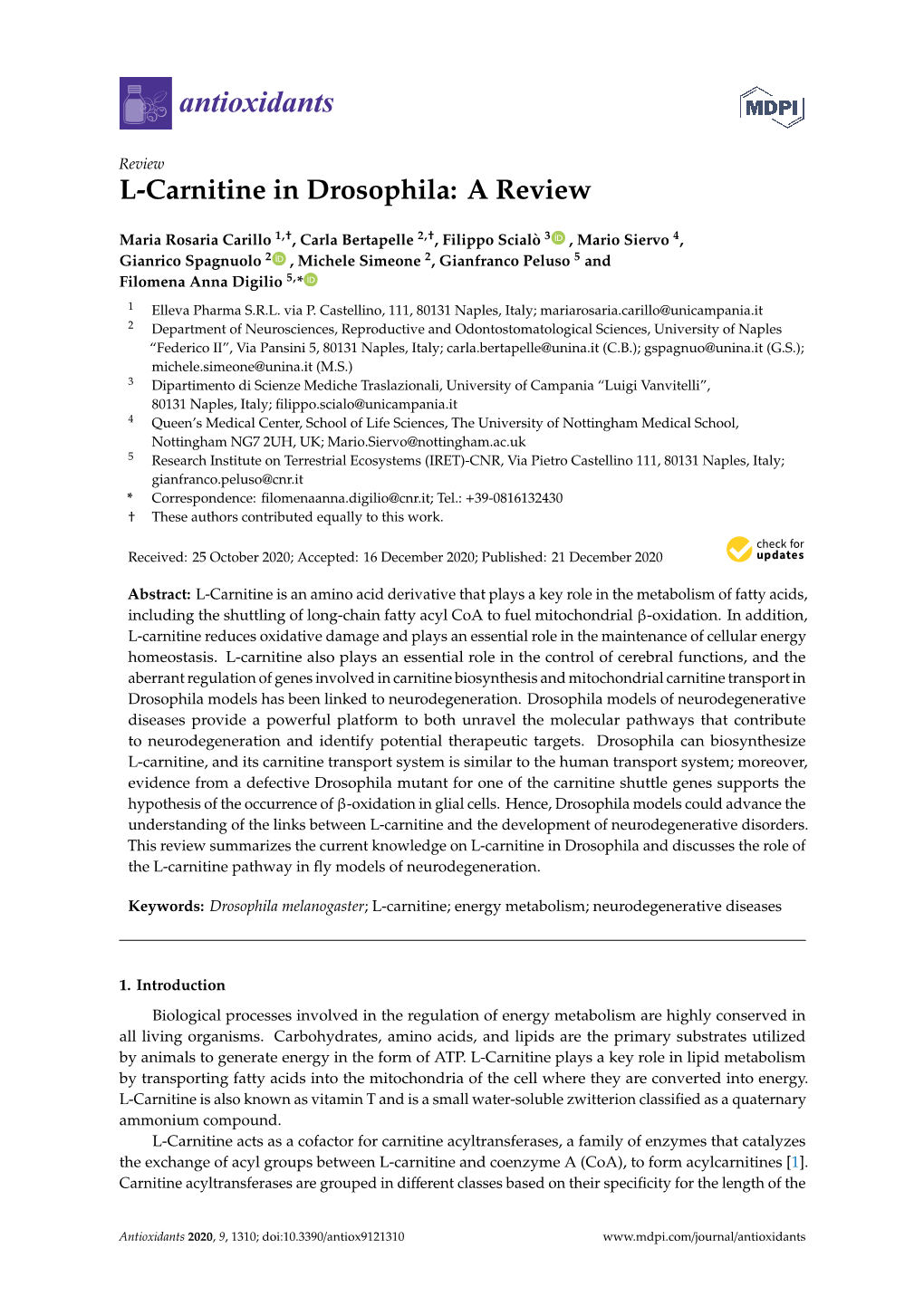
Load more
Recommended publications
-
A Common X-Linked Inborn Error of Carnitine Biosynthesis May Be a Risk Factor for Nondysmorphic Autism
A common X-linked inborn error of carnitine biosynthesis may be a risk factor for nondysmorphic autism Patrícia B. S. Celestino-Sopera,1, Sara Violanteb,c,1, Emily L. Crawfordd, Rui Luoe, Anath C. Lionelf, Elsa Delabyg, Guiqing Caih, Bekim Sadikovica, Kwanghyuk Leea, Charlene Loa, Kun Gaoe, Richard E. Persona, Timothy J. Mossa, Jennifer R. Germana, Ni Huangi, Marwan Shinawia,j,2, Diane Treadwell-Deeringj,k, Peter Szatmaril, Wendy Robertsm, Bridget Fernandezn, Richard J. Schroero, Roger E. Stevensono, Joseph D. Buxbaumh, Catalina Betancurg, Stephen W. Schererf,m, Stephan J. Sandersp, Daniel H. Geschwinde, James S. Sutcliffed, Matthew E. Hurlesi, Ronald J. A. Wandersb, Chad A. Shawa, Suzanne M. Leala, Edwin H. Cook, Jr.q, Robin P. Goin-Kochela,j,r, Frédéric M. Vazb,1, and Arthur L. Beaudeta,j,r,1,3 Departments of aMolecular and Human Genetics, kPsychiatry, and rPediatrics, Baylor College of Medicine, Houston, TX 77030; jTexas Children’s Hospital, Houston, TX 77030; bLaboratory Genetic Metabolic Disease, Departments of Clinical Chemistry and Pediatrics, Academic Medical Center, University of Amsterdam, 1105 AZ, Amsterdam, The Netherlands; cMetabolism and Genetics Group, Research Institute for Medicines and Pharmaceutical Sciences (iMed.UL), Faculdade de Farmácia, Universidade de Lisboa, 1649-003 Lisbon, Portugal; dDepartment of Molecular Physiology and Biophysics, Center for Molecular Neuroscience, Vanderbilt University, Nashville, TN 37232; eDepartment of Human Genetics, David Geffen School of Medicine, University of California, Los Angeles, -
The Capacity of Long-Term in Vitro Proliferation of Acute Myeloid
The Capacity of Long-Term in Vitro Proliferation of Acute Myeloid Leukemia Cells Supported Only by Exogenous Cytokines Is Associated with a Patient Subset with Adverse Outcome Annette K. Brenner, Elise Aasebø, Maria Hernandez-Valladares, Frode Selheim, Frode Berven, Ida-Sofie Grønningsæter, Sushma Bartaula-Brevik and Øystein Bruserud Supplementary Material S2 of S31 Table S1. Detailed information about the 68 AML patients included in the study. # of blasts Viability Proliferation Cytokine Viable cells Change in ID Gender Age Etiology FAB Cytogenetics Mutations CD34 Colonies (109/L) (%) 48 h (cpm) secretion (106) 5 weeks phenotype 1 M 42 de novo 241 M2 normal Flt3 pos 31.0 3848 low 0.24 7 yes 2 M 82 MF 12.4 M2 t(9;22) wt pos 81.6 74,686 low 1.43 969 yes 3 F 49 CML/relapse 149 M2 complex n.d. pos 26.2 3472 low 0.08 n.d. no 4 M 33 de novo 62.0 M2 normal wt pos 67.5 6206 low 0.08 6.5 no 5 M 71 relapse 91.0 M4 normal NPM1 pos 63.5 21,331 low 0.17 n.d. yes 6 M 83 de novo 109 M1 n.d. wt pos 19.1 8764 low 1.65 693 no 7 F 77 MDS 26.4 M1 normal wt pos 89.4 53,799 high 3.43 2746 no 8 M 46 de novo 26.9 M1 normal NPM1 n.d. n.d. 3472 low 1.56 n.d. no 9 M 68 MF 50.8 M4 normal D835 pos 69.4 1640 low 0.08 n.d. -
Analysis of Diet-Induced Differential Methylation, Expression, And
www.nature.com/scientificreports OPEN Analysis of diet-induced diferential methylation, expression, and interactions of lncRNA and protein- Received: 2 March 2018 Accepted: 29 June 2018 coding genes in mouse liver Published: xx xx xxxx Jose P. Silva1 & Derek van Booven2 Long non-coding RNAs (lncRNAs) regulate expression of protein-coding genes in cis through chromatin modifcations including DNA methylation. Here we interrogated whether lncRNA genes may regulate transcription and methylation of their fanking or overlapping protein-coding genes in livers of mice exposed to a 12-week cholesterol-rich Western-style high fat diet (HFD) relative to a standard diet (STD). Deconvolution analysis of cell type-specifc marker gene expression suggested similar hepatic cell type composition in HFD and STD livers. RNA-seq and validation by nCounter technology revealed diferential expression of 14 lncRNA genes and 395 protein-coding genes enriched for functions in steroid/cholesterol synthesis, fatty acid metabolism, lipid localization, and circadian rhythm. While lncRNA and protein-coding genes were co-expressed in 53 lncRNA/protein-coding gene pairs, both were diferentially expressed only in 4 lncRNA/protein-coding gene pairs, none of which included protein- coding genes in overrepresented pathways. Furthermore, 5-methylcytosine DNA immunoprecipitation sequencing and targeted bisulfte sequencing revealed no diferential DNA methylation of genes in overrepresented pathways. These results suggest lncRNA/protein-coding gene interactions in cis play a minor role mediating hepatic expression of lipid metabolism/localization and circadian clock genes in response to chronic HFD feeding. More than 70% of the mammalian genome is transcribed as non-coding RNA (ncRNA) while only 1–2% of the mammalian genome is transcribed as protein-coding RNA1–3. -
A Class of Circadian Long Non-Coding Rnas Mark Enhancers Modulating Long-Range Circadian Gene Regulation Zenghua Fan1,2, Meng Zhao3, Parth D
5720–5738 Nucleic Acids Research, 2017, Vol. 45, No. 10 Published online 8 March 2017 doi: 10.1093/nar/gkx156 A class of circadian long non-coding RNAs mark enhancers modulating long-range circadian gene regulation Zenghua Fan1,2, Meng Zhao3, Parth D. Joshi4,PingLi5, Yan Zhang5, Weimin Guo3, Yichi Xu1,2, Haifang Wang3, Zhihu Zhao5 and Jun Yan3,* 1 CAS-MPG Partner Institute for Computational Biology, Shanghai Institutes for Biological Sciences, Chinese Downloaded from https://academic.oup.com/nar/article-abstract/45/10/5720/3063381 by guest on 06 March 2019 Academy of Sciences, 320 Yue Yang Road, Shanghai 200031, China, 2University of Chinese Academy of Sciences, Shanghai 200031, China, 3Institute of Neuroscience, State Key Laboratory of Neuroscience, CAS Center for Excellence in Brain Science and Intelligence Technology, Shanghai Institutes for Biological Sciences, Chinese Academy of Sciences, Shanghai 200031, China, 4Department of Genes and Behavior, Max Planck Institute for Biophysical Chemistry, Am Fassberg 11, 37077 Gottingen,¨ Germany and 5Beijing Institute of Biotechnology, 20 Dongdajie Street, Fengtai District, Beijing 100071, China Received February 04, 2017; Editorial Decision February 23, 2017; Accepted February 24, 2017 ABSTRACT regulation and shed new lights on the evolutionary origin of lncRNAs. Circadian rhythm exerts its influence on animal physiology and behavior by regulating gene expres- sion at various levels. Here we systematically ex- INTRODUCTION plored circadian long non-coding RNAs (lncRNAs) Circadian rhythm is an intrinsic 24 h oscillation of var- in mouse liver and examined their circadian reg- ious physiological processes and behaviors synchronized ulation. We found that a significant proportion of with daily light/dark cycle in a wide-range of species. -
An Overview of Ascorbic Acid Biochemistry
Ankara Ecz. Fak. Derg. J. Fac. Pharm, Ankara 38 (3) 233-255, 2009 38 (3) 233-255, 2009 AN OVERVIEW OF ASCORBIC ACID BIOCHEMISTRY ASKORBĐK ASĐT BĐYOKĐMYASINA BĐR BAKIŞ Aysun HACIŞEVKĐ Gazi University, Faculty of Pharmacy, Department of Biochemistry, 06330 Etiler-Ankara, TURKEY ABSTRACT Ascorbic acid (vitamin C) is a water-soluble micronutrient required for multiple biological functions. Ascorbic acid is a cofactor for several enzymes participating in the post-translational hydroxylation of collagen, in the biosynthesis of carnitine, in the conversion of the neurotransmitter dopamine to norepinephrine, in peptide amidation and in tyrosine metabolism. In addition, vitamin C is an important regulator of iron uptake, It reduces ferric Fe 3+ to ferrous Fe 2+ ions, thus promoting dietary non-haem iron absorption from the gastrointestinal tract, and stabilizes iron-binding proteins. Most animals are able to synthesise vitamin C from glucose, but humans, other primates, guinea pigs and fruit bats lack the last enzyme involved in the synthesis of vitamin C (gulonolactone oxidase) and so require the presence of the vitamin in their diet. Thus the prolonged deprivation of vitamin C generates defects in the post-translational modification of collagen that cause scurvy and eventually death. In addition to its antiscorbutic action, vitamin C is a potent reducing agent and scavenger of free radicals in biological systems. Key words: Ascorbic acid, Vitamin C, Antioxidant, Oxidative stress ÖZET Askorbik asit, suda çözünen çoklu biyolojik fonksiyonları olan bir mikrobesindir. Kollajen hidroksilasyonu, karnitin biyosentezi, dopaminin norepinefrine çevrimi, peptid amidasyonu ve tirozin metabolizmasına katılan çeşitli enzimler için bir kofaktördür. Ayrıca vitamin C demir alımında önemli bir regülatördür, ferrik demiri ferro formuna redükler ve böylece gastrointestinal sistemden diyet nonhem demir absorbsiyonunu sağlar ve demir bağlı proteinleri stabilize eder. -
Downloaded from the App Store and Nucleobase, Nucleotide and Nucleic Acid Metabolism 7 Google Play
Hoytema van Konijnenburg et al. Orphanet J Rare Dis (2021) 16:170 https://doi.org/10.1186/s13023-021-01727-2 REVIEW Open Access Treatable inherited metabolic disorders causing intellectual disability: 2021 review and digital app Eva M. M. Hoytema van Konijnenburg1†, Saskia B. Wortmann2,3,4†, Marina J. Koelewijn2, Laura A. Tseng1,4, Roderick Houben6, Sylvia Stöckler‑Ipsiroglu5, Carlos R. Ferreira7 and Clara D. M. van Karnebeek1,2,4,8* Abstract Background: The Treatable ID App was created in 2012 as digital tool to improve early recognition and intervention for treatable inherited metabolic disorders (IMDs) presenting with global developmental delay and intellectual disabil‑ ity (collectively ‘treatable IDs’). Our aim is to update the 2012 review on treatable IDs and App to capture the advances made in the identifcation of new IMDs along with increased pathophysiological insights catalyzing therapeutic development and implementation. Methods: Two independent reviewers queried PubMed, OMIM and Orphanet databases to reassess all previously included disorders and therapies and to identify all reports on Treatable IDs published between 2012 and 2021. These were included if listed in the International Classifcation of IMDs (ICIMD) and presenting with ID as a major feature, and if published evidence for a therapeutic intervention improving ID primary and/or secondary outcomes is avail‑ able. Data on clinical symptoms, diagnostic testing, treatment strategies, efects on outcomes, and evidence levels were extracted and evaluated by the reviewers and external experts. The generated knowledge was translated into a diagnostic algorithm and updated version of the App with novel features. Results: Our review identifed 116 treatable IDs (139 genes), of which 44 newly identifed, belonging to 17 ICIMD categories. -
Potential Role of L-Carnitine in Autism Spectrum Disorder
Journal of Clinical Medicine Review Potential Role of L-Carnitine in Autism Spectrum Disorder Alina K˛epka 1,† , Agnieszka Ochoci ´nska 1,*,† , Sylwia Chojnowska 2 , Małgorzata Borzym-Kluczyk 3, Ewa Skorupa 1, Małgorzata Kna´s 2 and Napoleon Waszkiewicz 4 1 Department of Biochemistry, Radioimmunology and Experimental Medicine, The Children’s Memorial Health Institute, 04-730 Warsaw, Poland; [email protected] (A.K.); [email protected] (E.S.) 2 Faculty of Health Sciences, Lomza State University of Applied Sciences, 18-400 Lomza, Poland; [email protected] (S.C.); [email protected] (M.K.) 3 Department of Pharmaceutical Biochemistry, Medical University of Bialystok, 15-089 Bialystok, Poland; [email protected] 4 Department of Psychiatry, Medical University of Bialystok, 15-089 Bialystok, Poland; [email protected] * Correspondence: [email protected]; Tel.: +48-22-815-73-01 † These authors are sharing the first place. Both contributed equally to this work. Abstract: L-carnitine plays an important role in the functioning of the central nervous system, and especially in the mitochondrial metabolism of fatty acids. Altered carnitine metabolism, abnormal fatty acid metabolism in patients with autism spectrum disorder (ASD) has been documented. ASD is a complex heterogeneous neurodevelopmental condition that is usually diagnosed in early child- hood. Patients with ASD require careful classification as this heterogeneous clinical category may include patients with an intellectual disability or high functioning, epilepsy, language impairments, or associated Mendelian genetic conditions. L-carnitine participates in the long-chain oxidation of fatty acids in the brain, stimulates acetylcholine synthesis (donor of the acyl groups), stimulates ex- pression of growth-associated protein-43, prevents cell apoptosis and neuron damage and stimulates Citation: K˛epka,A.; Ochoci´nska,A.; neurotransmission. -
A High-Throughput Approach to Uncover Novel Roles of APOBEC2, a Functional Orphan of the AID/APOBEC Family
Rockefeller University Digital Commons @ RU Student Theses and Dissertations 2018 A High-Throughput Approach to Uncover Novel Roles of APOBEC2, a Functional Orphan of the AID/APOBEC Family Linda Molla Follow this and additional works at: https://digitalcommons.rockefeller.edu/ student_theses_and_dissertations Part of the Life Sciences Commons A HIGH-THROUGHPUT APPROACH TO UNCOVER NOVEL ROLES OF APOBEC2, A FUNCTIONAL ORPHAN OF THE AID/APOBEC FAMILY A Thesis Presented to the Faculty of The Rockefeller University in Partial Fulfillment of the Requirements for the degree of Doctor of Philosophy by Linda Molla June 2018 © Copyright by Linda Molla 2018 A HIGH-THROUGHPUT APPROACH TO UNCOVER NOVEL ROLES OF APOBEC2, A FUNCTIONAL ORPHAN OF THE AID/APOBEC FAMILY Linda Molla, Ph.D. The Rockefeller University 2018 APOBEC2 is a member of the AID/APOBEC cytidine deaminase family of proteins. Unlike most of AID/APOBEC, however, APOBEC2’s function remains elusive. Previous research has implicated APOBEC2 in diverse organisms and cellular processes such as muscle biology (in Mus musculus), regeneration (in Danio rerio), and development (in Xenopus laevis). APOBEC2 has also been implicated in cancer. However the enzymatic activity, substrate or physiological target(s) of APOBEC2 are unknown. For this thesis, I have combined Next Generation Sequencing (NGS) techniques with state-of-the-art molecular biology to determine the physiological targets of APOBEC2. Using a cell culture muscle differentiation system, and RNA sequencing (RNA-Seq) by polyA capture, I demonstrated that unlike the AID/APOBEC family member APOBEC1, APOBEC2 is not an RNA editor. Using the same system combined with enhanced Reduced Representation Bisulfite Sequencing (eRRBS) analyses I showed that, unlike the AID/APOBEC family member AID, APOBEC2 does not act as a 5-methyl-C deaminase. -
Non-Recurrent MECP2 Duplications Mediated by Genomic Architecture-Driven
Downloaded from genome.cshlp.org on October 4, 2021 - Published by Cold Spring Harbor Laboratory Press 1 Non-recurrent MECP2 duplications mediated by genomic architecture-driven DNA breaks and break-induced replication repair Running title: Genomics of MECP2 duplications Marijke Bauters,1,2,1 Hilde Van Esch,3,1 Michael %. Frie(,4 Odile Boespflug- ,anguy,5 Martin .enker,6 Angela M. Vianna-Morgante,0 1arla 2osenberg,0 %aakko Ignatius,8 Martine 2aynaud,5 6aren Hollanders,1,2 6aren 7ovaerts,1,2 6ris Vandenreijt,1,2 Florence Niel,5 8ierre Blanc,5 2oger E. Stevenson,4 %ean-8ierre Fryns,3 8eter Marynen,1,2 1harles E. Schwart(,4 and 7uy Froyen1,2,11 1Human Genome Laboratory, Dept. for Molecular and Developmental Genetics, IB, Leuven, Belgium$ 2Human Genome Laboratory, Dept. of Human Genetics, %.U.Leuven, Leuven, Belgium$ 3Dept. of Human Genetics, University Hospital Gasthuisberg, Leuven, Belgium$ 4JC Self ,esearch Institute of Human Genetics, Greenwood Genetic Center, Greenwood, SC$ .Centre Hospitalier Universitaire, Clermont-0D, G1n1ti2ue Humaine, Clermont-0errand, 0rance$ 3Institute of Human Genetics, University of Erlangen- Nuremberg, Erlangen, Germany$ 7Dept. Genetics and Evolutionary Biology, Institute of Biosciences, University of S6o Paulo, Bra7il$ 8Dept. Clinical Genetics, Oulu University Hospital and Oulu University, 0inland$ 9Centre Hospitalier Universitaire de Tours, Service de G1n1ti2ue, Tours, 0rance Ke words: MECP2, duplication, recombination model, breakpoint anal sis 1 ,hese two authors contributed equally to this work. Bauters_ms4.doc 14-3-2008 Downloaded from genome.cshlp.org on October 4, 2021 - Published by Cold Spring Harbor Laboratory Press 2 111orresponding author. E-mail guy.froyen@ med.kuleuven.be; fax: +32-16-340166 Address for correspondence: Dr. -
2012 Portfolio Analysis Projects: Question 2: How Can I Understand
2012 Portfolio Analysis Projects Please note that data are not yet final; additional projects may be added. QUESTION 2: HOW CAN I UNDERSTAND WHAT IS HAPPENING? 2.S.A Support at least four research projects to identify mechanisms of fever, metabolic and/or immune system interactions with the central nervous system that may influence ASD during prenatal-postnatal life by 2010. IACC Recommended Budget: $9,800,000 over 4 years. (Fever studies to be started by 2012.) Principal Project Title Institution Funding Funder Investigator Systematic characterization of the Alaedini, Weill Cornell Medical $0.00 Department immune response to gluten and Armin College of Defense casein in autism spectrum disorders Autoimmunity against novel antigens Balice- University of $320,000. National in neuropsychiatric dysfunction Gordon, Pennsylvania 00 Institutes of Rita Health Convergence of immune and genetic Barrow, University of $0.00 Brain & signaling pathways in autism and Stephanie California, Davis Behavior schizophrenia Research Foundation Altered placental tryptophan Bonnin, University of $535,699. Department metabolism: A crucial molecular Alexandre Southern California 00 of Defense pathway for the fetal programming of neurodevelopmental disorders Brain mitochondrial abnormalities in Chauhan, New York State $20,000.0 Autism autism Abha Institute for Basic 0 Research Research in Institute Developmental Disabilities Mechanisms of synaptic alterations in Dunaevsky University of $579,882. Department a neuroinflammation model of autism , Anna Nebraska Medical -
Analysis of Carnitine and Acylcarnitines in Biological Fluids and Application to a Clinical Study
ANALYSIS OF CARNITINE AND ACYLCARNITINES IN BIOLOGICAL FLUIDS AND APPLICATION TO A CLINICAL STUDY Inauguraldissertation zur Erlangung der Würde eines Doktors der Philosophie vorgelegt der Philosophisch-Naturwissenschaftlichen Fakultät der Universität Basel von Laurence Vernez aus Villars-Bramard, VD Genf, 2005 Genehmigt von der Philosophisch-Naturwissenschaftlichen Fakultät auf Antrag von: Prof. Dr. Stephan Krähenbühl Prof. Dr. Gérard Hopfgartner Prof. Dr. Wolfgang Thormann Basel, den 6. April 2004 Prof. Dr. Marcel Tanner Dekan POUR TOI QUI M’ATTENDS ENCORE Un savant dans son laboratoire n’est pas seulement un technicien: c’est aussi un enfant placé en face de phénomènes naturels qui l’impressionnent comme un conte de fées Marie Curie-Sklodowska, 1933 Table of contents TABLE OF CONTENTS Résumé 13 Zusammenfassung 16 Summary 18 List of abbreviations 21 1. Introduction 23 1.1 General aspects 23 1.2 Carnitine functions 25 1.2.1. Mitochondrial long-chain fatty acid oxidation 25 1.2.2. Buffering of the mitochondrial acyl-CoA/CoA ratio 27 1.2.3. Removal of potentially toxic acyl-groups 27 1.2.4. Fatty acids oxidation in peroxisomes 28 1.3 Carnitine biosynthesis 28 1.4 Carnitine homeostasis 31 1.4.1. Absorption 31 1.4.2. Tissue distribution - carnitine transporters 32 i. Regulation of tissue distribution 33 ii. Kinetic of exogenous carnitine 33 1.4.3. Metabolism 34 1.4.4. Elimination - role of kidney 35 1.5 Carnitine deficiency 36 1.5.1. Primary carnitine deficiency 37 i. Systemic carnitine deficiency (SCD) 37 ii. Muscle carnitine deficiency (MCD) 38 1.5.2. Secondary carnitine deficiency 38 i. -
Characterizing Genomic Duplication in Autism Spectrum Disorder by Edward James Higginbotham a Thesis Submitted in Conformity
Characterizing Genomic Duplication in Autism Spectrum Disorder by Edward James Higginbotham A thesis submitted in conformity with the requirements for the degree of Master of Science Graduate Department of Molecular Genetics University of Toronto © Copyright by Edward James Higginbotham 2020 i Abstract Characterizing Genomic Duplication in Autism Spectrum Disorder Edward James Higginbotham Master of Science Graduate Department of Molecular Genetics University of Toronto 2020 Duplication, the gain of additional copies of genomic material relative to its ancestral diploid state is yet to achieve full appreciation for its role in human traits and disease. Challenges include accurately genotyping, annotating, and characterizing the properties of duplications, and resolving duplication mechanisms. Whole genome sequencing, in principle, should enable accurate detection of duplications in a single experiment. This thesis makes use of the technology to catalogue disease relevant duplications in the genomes of 2,739 individuals with Autism Spectrum Disorder (ASD) who enrolled in the Autism Speaks MSSNG Project. Fine-mapping the breakpoint junctions of 259 ASD-relevant duplications identified 34 (13.1%) variants with complex genomic structures as well as tandem (193/259, 74.5%) and NAHR- mediated (6/259, 2.3%) duplications. As whole genome sequencing-based studies expand in scale and reach, a continued focus on generating high-quality, standardized duplication data will be prerequisite to addressing their associated biological mechanisms. ii Acknowledgements I thank Dr. Stephen Scherer for his leadership par excellence, his generosity, and for giving me a chance. I am grateful for his investment and the opportunities afforded me, from which I have learned and benefited. I would next thank Drs.